Ultrafast piezocapacitive soft pressure sensors with over 10 kHz bandwidth via bonded microstructured interfaces
- PMID: 38589497
- PMCID: PMC11001880
- DOI: 10.1038/s41467-024-47408-z
Ultrafast piezocapacitive soft pressure sensors with over 10 kHz bandwidth via bonded microstructured interfaces
Abstract
Flexible pressure sensors can convert mechanical stimuli to electrical signals to interact with the surroundings, mimicking the functionality of the human skins. Piezocapacitive pressure sensors, a class of most widely used devices for artificial skins, however, often suffer from slow response-relaxation speed (tens of milliseconds) and thus fail to detect dynamic stimuli or high-frequency vibrations. Here, we show that the contact-separation behavior of the electrode-dielectric interface is an energy dissipation process that substantially determines the response-relaxation time of the sensors. We thus reduce the response and relaxation time to ~0.04 ms using a bonded microstructured interface that effectively diminishes interfacial friction and energy dissipation. The high response-relaxation speed allows the sensor to detect vibrations over 10 kHz, which enables not only dynamic force detection, but also acoustic applications. This sensor also shows negligible hysteresis to precisely track dynamic stimuli. Our work opens a path that can substantially promote the response-relaxation speed of piezocapacitive pressure sensors into submillisecond range and extend their applications in acoustic range.
© 2024. The Author(s).
Conflict of interest statement
The authors declare no competing interests.
Figures
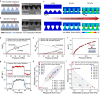
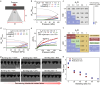
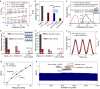
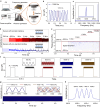
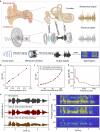
Similar articles
-
Microstructured Polyelectrolyte Elastomer-Based Ionotronic Sensors with High Sensitivities and Excellent Stability for Artificial Skins.Adv Mater. 2024 Mar;36(11):e2310429. doi: 10.1002/adma.202310429. Epub 2023 Dec 17. Adv Mater. 2024. PMID: 38095237
-
A Flexible Piezocapacitive Pressure Sensor with Microsphere-Array Electrodes.Nanomaterials (Basel). 2023 May 23;13(11):1702. doi: 10.3390/nano13111702. Nanomaterials (Basel). 2023. PMID: 37299605 Free PMC article.
-
Highly Sensitive Piezocapacitive Sensor for Detecting Static and Dynamic Pressure Using Ion-Gel Thin Films and Conductive Elastomeric Composites.ACS Appl Mater Interfaces. 2017 Oct 18;9(41):36206-36219. doi: 10.1021/acsami.7b11700. Epub 2017 Oct 6. ACS Appl Mater Interfaces. 2017. PMID: 28984435
-
Flexible microstructured pressure sensors: design, fabrication and applications.Nanotechnology. 2022 May 20;33(32). doi: 10.1088/1361-6528/ac6812. Nanotechnology. 2022. PMID: 35439735 Review.
-
Advancements in MXene-based composites for electronic skins.J Mater Chem B. 2024 Jan 24;12(4):895-915. doi: 10.1039/d3tb02247a. J Mater Chem B. 2024. PMID: 38194290 Review.
Cited by
-
Self-powered visualized tactile-acoustic sensor for accurate artificial perception with high brightness and record-low detection limit.Sci Adv. 2024 Nov;10(44):eadq8989. doi: 10.1126/sciadv.adq8989. Epub 2024 Oct 30. Sci Adv. 2024. PMID: 39475613 Free PMC article.
-
An ultrasensitive multimodal intracranial pressure biotelemetric system enabled by exceptional point and iontronics.Nat Commun. 2024 Nov 5;15(1):9557. doi: 10.1038/s41467-024-53836-8. Nat Commun. 2024. PMID: 39500903 Free PMC article.
-
Flexible Sensors Based on Conductive Polymer Composites.Sensors (Basel). 2024 Jul 18;24(14):4664. doi: 10.3390/s24144664. Sensors (Basel). 2024. PMID: 39066060 Free PMC article. Review.
References
-
- Huang Y-C, et al. Sensitive pressure sensors based on conductive microstructured air-gap gates and two-dimensional semiconductor transistors. Nat. Electron. 2020;3:59–69. doi: 10.1038/s41928-019-0356-5. - DOI
LinkOut - more resources
Full Text Sources