Force Spectroscopy on Single Molecules of Life
- PMID: 32478214
- PMCID: PMC7254507
- DOI: 10.1021/acsomega.0c00814
Force Spectroscopy on Single Molecules of Life
Abstract
Biomolecules such as nucleic acids and proteins constitute the cells and its organelles that form the crucial components in all living organisms. They are associated with a variety of cellular processes during which they undergo conformational orientations. The structural rearrangements resulting from protein-protein, protein-DNA, and protein-drug interactions vary in spatial and temporal length scales. Force is one of the important key factors which regulate these interactions. The magnitude of the force can vary from sub-piconewtons to several thousands of piconewtons. Single-molecule force spectroscopy acts as a powerful tool which is capable of investigating mechanical stability and conformational rearrangements arising in biomolecules due to the above interactions. Real-time observation of conformational dynamics including access to rare or transient states and the estimation of mean dwell times using these tools aids in the kinetic analysis of these interactions. In this review, we highlight the capabilities of common force spectroscopy techniques such as optical tweezers, magnetic tweezers, and atomic force microscopy with case studies on emerging applications.
Copyright © 2020 American Chemical Society.
Conflict of interest statement
The author declares no competing financial interest.
Figures
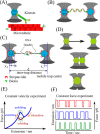
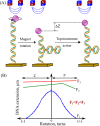
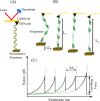
Similar articles
-
An Introduction to Magnetic Tweezers.Methods Mol Biol. 2024;2694:375-401. doi: 10.1007/978-1-0716-3377-9_18. Methods Mol Biol. 2024. PMID: 37824014
-
Interrogating biology with force: single molecule high-resolution measurements with optical tweezers.Biophys J. 2013 Sep 17;105(6):1293-303. doi: 10.1016/j.bpj.2013.08.007. Biophys J. 2013. PMID: 24047980 Free PMC article. Review.
-
Single-molecule force spectroscopy using the NanoTracker optical tweezers platform: from design to application.Curr Pharm Biotechnol. 2009 Aug;10(5):467-73. doi: 10.2174/138920109788922164. Curr Pharm Biotechnol. 2009. PMID: 19689314
-
Probing the mechanical properties, conformational changes, and interactions of nucleic acids with magnetic tweezers.J Struct Biol. 2017 Jan;197(1):26-36. doi: 10.1016/j.jsb.2016.06.022. Epub 2016 Jun 29. J Struct Biol. 2017. PMID: 27368129 Review.
-
Recent Advances in Biological Single-Molecule Applications of Optical Tweezers and Fluorescence Microscopy.Methods Enzymol. 2017;582:85-119. doi: 10.1016/bs.mie.2016.09.047. Epub 2016 Dec 12. Methods Enzymol. 2017. PMID: 28062046
Cited by
-
Insight into Single-Molecule Imaging Techniques for the Study of Prokaryotic Genome Maintenance.Chem Biomed Imaging. 2024 Jun 18;2(9):595-614. doi: 10.1021/cbmi.4c00037. eCollection 2024 Sep 23. Chem Biomed Imaging. 2024. PMID: 39328428 Free PMC article. Review.
-
Multi-parameter photon-by-photon hidden Markov modeling.Nat Commun. 2022 Feb 22;13(1):1000. doi: 10.1038/s41467-022-28632-x. Nat Commun. 2022. PMID: 35194038 Free PMC article.
-
Advances in the Biological Application of Force-Induced Remnant Magnetization Spectroscopy.Molecules. 2022 Mar 23;27(7):2072. doi: 10.3390/molecules27072072. Molecules. 2022. PMID: 35408471 Free PMC article. Review.
-
Development of Single Molecule Techniques for Sensing and Manipulation of CRISPR and Polymerase Enzymes.Small. 2023 Sep;19(38):e2300328. doi: 10.1002/smll.202300328. Epub 2023 May 24. Small. 2023. PMID: 37226388 Free PMC article. Review.
-
Optical Monitoring of In Situ Iron Loading into Single, Native Ferritin Proteins.Nano Lett. 2023 Apr 26;23(8):3251-3258. doi: 10.1021/acs.nanolett.3c00042. Epub 2023 Apr 13. Nano Lett. 2023. PMID: 37053043 Free PMC article.
References
-
- Los G. V.; Encell L. P.; McDougall M. G.; Hartzell D. D.; Karassina N.; Zimprich C.; Wood M. G.; Learish R.; Ohana R. F.; Urh M.; Simpson D.; Mendez J.; Zimmerman K.; Otto P.; Vidugiris G.; Zhu J.; Darzins A.; Klaubert D. H.; Bulleit R. F.; Wood K. V. HaloTag: A novel protein labelling technology for cell imaging and protein analysis. ACS Chem. Biol. 2008, 3, 373–382. 10.1021/cb800025k. - DOI - PubMed
Publication types
LinkOut - more resources
Full Text Sources