Electron tunneling of hierarchically structured silver nanosatellite particles for highly conductive healable nanocomposites
- PMID: 32382034
- PMCID: PMC7206115
- DOI: 10.1038/s41467-020-15709-8
Electron tunneling of hierarchically structured silver nanosatellite particles for highly conductive healable nanocomposites
Abstract
Healable conductive materials have received considerable attention. However, their practical applications are impeded by low electrical conductivity and irreversible degradation after breaking/healing cycles. Here we report a highly conductive completely reversible electron tunneling-assisted percolation network of silver nanosatellite particles for putty-like moldable and healable nanocomposites. The densely and uniformly distributed silver nanosatellite particles with a bimodal size distribution are generated by the radical and reactive oxygen species-mediated vigorous etching and reduction reaction of silver flakes using tetrahydrofuran peroxide in a silicone rubber matrix. The close work function match between silicone and silver enables electron tunneling between nanosatellite particles, increasing electrical conductivity by ~5 orders of magnitude (1.02×103 Scm-1) without coalescence of fillers. This results in ~100% electrical healing efficiency after 1000 breaking/healing cycles and stability under water immersion and 6-month exposure to ambient air. The highly conductive moldable nanocomposite may find applications in improvising and healing electrical parts.
Conflict of interest statement
The authors declare no competing interests.
Figures
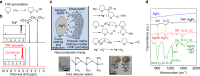
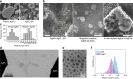
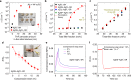
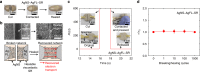
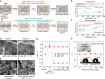
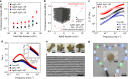
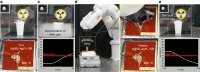
Similar articles
-
Invariable resistance of conductive nanocomposite over 30% strain.Sci Adv. 2022 Aug 12;8(32):eabn3365. doi: 10.1126/sciadv.abn3365. Epub 2022 Aug 12. Sci Adv. 2022. PMID: 35960794 Free PMC article.
-
Highly Conductive Strong Healable Nanocomposites via Diels-Alder Reaction and Filler-Polymer Covalent Bifunctionalization.Small. 2022 Jan;18(3):e2104764. doi: 10.1002/smll.202104764. Epub 2021 Nov 10. Small. 2022. PMID: 34761523
-
Highly conductive thermoresponsive silver nanowire PNIPAM nanocomposite for reversible electrical switch.Soft Matter. 2022 Sep 28;18(37):7171-7180. doi: 10.1039/d2sm00700b. Soft Matter. 2022. PMID: 36098069
-
A healable, semitransparent silver nanowire-polymer composite conductor.Adv Mater. 2013 Aug 14;25(30):4186-91. doi: 10.1002/adma.201301069. Epub 2013 Jun 24. Adv Mater. 2013. PMID: 23794459
-
High-performance stretchable conductive nanocomposites: materials, processes, and device applications.Chem Soc Rev. 2019 Mar 18;48(6):1566-1595. doi: 10.1039/c8cs00706c. Chem Soc Rev. 2019. PMID: 30519703 Review.
Cited by
-
Reversible electrical percolation in a stretchable and self-healable silver-gradient nanocomposite bilayer.Nat Commun. 2022 Sep 5;13(1):5233. doi: 10.1038/s41467-022-32966-x. Nat Commun. 2022. PMID: 36064549 Free PMC article.
-
Flexible Sensors Based on Conductive Polymer Composites.Sensors (Basel). 2024 Jul 18;24(14):4664. doi: 10.3390/s24144664. Sensors (Basel). 2024. PMID: 39066060 Free PMC article. Review.
-
Autonomous self-healing supramolecular polymer transistors for skin electronics.Nat Commun. 2024 Apr 23;15(1):3433. doi: 10.1038/s41467-024-47718-2. Nat Commun. 2024. PMID: 38653966 Free PMC article.
-
Modeling of Electrical Conductivity for Polymer-Carbon Nanofiber Systems.Materials (Basel). 2022 Oct 10;15(19):7041. doi: 10.3390/ma15197041. Materials (Basel). 2022. PMID: 36234382 Free PMC article.
-
Invariable resistance of conductive nanocomposite over 30% strain.Sci Adv. 2022 Aug 12;8(32):eabn3365. doi: 10.1126/sciadv.abn3365. Epub 2022 Aug 12. Sci Adv. 2022. PMID: 35960794 Free PMC article.
References
Grants and funding
LinkOut - more resources
Full Text Sources
Other Literature Sources