Irving Langmuir, the General Electric Research Laboratory, and when applications lead to theory
To this point, I’ve written tens of thousands of words on this Substack covering the work of scientists from the early-to-mid-1900s — a golden era of American science and innovation — in a familiar setting: the university. But, in fact, the university is not the setting in which I first came to know most of these famous scientists. I initially read about most of these researchers in books like The Making of the Atomic Bomb and other histories which cover the work (and shockingly fun lives) of these scientists largely in applied settings.
Almost all of them did some form of extremely applied work at some point in their career. For many, this consisted of one or two stints doing applied research for the military during World Wars I and II. If they were lucky, they were doing scientific work, but many would even find themselves doing statistics for some military department. Even for those who did not serve in the military, it was often not possible to avoid doing applied work. Until about 1952, a very large proportion of university funding came from industry and state governments rather than the federal government. And these groups, as funders, had practical requirements for how the funding was to be deployed. So, most scientists had to play nicely and do practical (or practical-adjacent) work a large percentage of the time whether they wanted to or not. That’s just the way it was.
One theory I hold that is not backed by extremely compelling statistical evidence is that the pervasiveness of applied research experiences in the early 1900s was great for the scientists and the innovation ecosystem as a whole. These detours, even if they were responsible for the scientists being temporarily underemployed, were, I believe, super-additive. In reading the historical accounts of the period, it is clear that many scientists — possibly most — had their research and research problems shaped by their applied experiences in one way or another.
Of course, one could argue that these great researchers would have been even more productive had they not been wasting their time working on practical problems. And I really couldn’t rigorously refute that claim. This general system of how things worked applied more or less equally to an entire generation’s scientific culture altogether in America, and the system later changed for the next generation altogether. So, there is no cute natural experiment to tease apart the effect of these applied experiences on the community as a whole.
But that, on its own, does not shake my belief in the super-additivity of applied research on early-to-mid 20th-century researchers and their outstanding outputs.
In this week’s post, I hope to help you understand why I see it this way. I am going to tell you the story of Irving Langmuir and how exactly he got started on the course of research that would win him a Nobel Prize. In the process, I hope you come to see just how effective the ‘constraint’ of industrial usefulness can be — even for basic researchers.
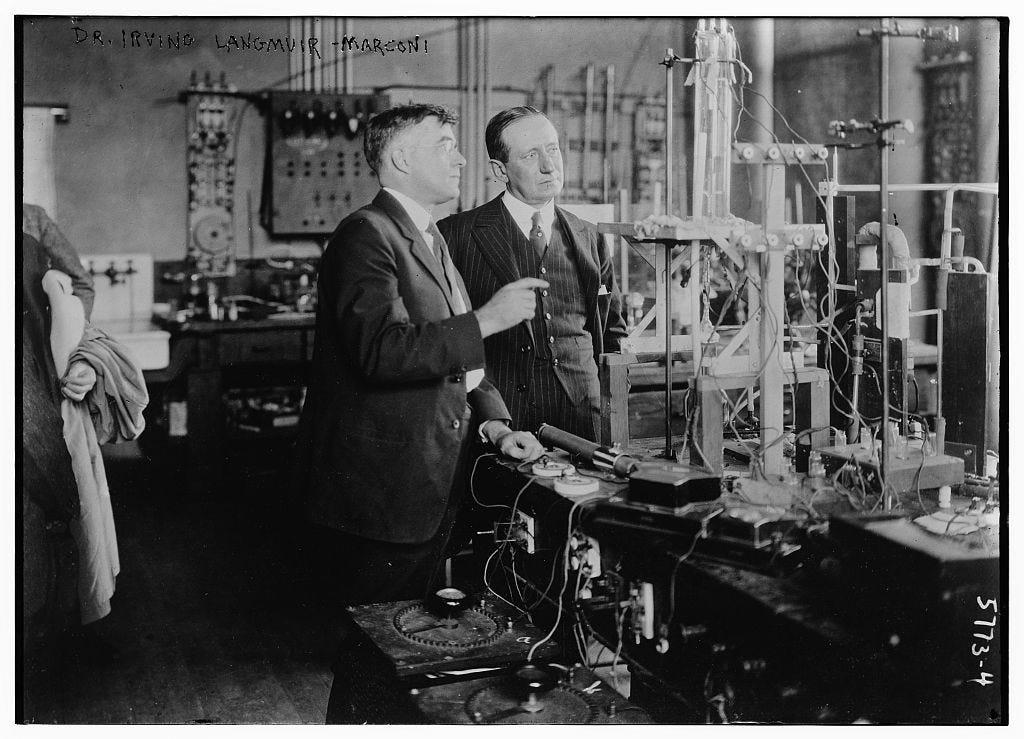
Shout out to Jordan Schneider for the book recommendation that inspired this piece. Subscribe to his ChinaTalk Substack or podcast to learn all about current Chinese tariffs (and more).
The book much of this piece draws from is called From Know-How to Nowhere by Elting Morison, a remarkably out-of-print book about the massive importance of learning by doing in the history of American innovation.1
This post is another in partnership with the Good Science Project.
The birth of the lab
Irving Langmuir conducted his research out of an old barn in Schenectady, New York. That barn was called the General Electric Research Laboratory. How exactly did this barn grow into a powerhouse of applied and basic research — generating multi-billion dollar ideas and a Nobel Prize? Let me set the scene.
In 1900, the light bulb was still in its early stages. It was bad, but 24 million were sold in 1900 alone in spite of this — and the market was rapidly growing. The bulbs’ main technical problems were that they could not sustain a constant level of light, they burned out after a short period, the interior walls blackened, and they used a relatively high level of electric current.
Attempts were underway all over Europe to overcome these issues. Morison writes:
There were constant attempts to improve it and some progress. The first lamps, in 1880, used about 6 watts per candle (a candle is a unit of luminous intensity, being the light emitted by five square millimeters of platinum at the temperature of solidification). By 1900, the lamps were down to 3.1 watts per candle. But since it had been figured that a source of white light of perfect efficiency should consume something less than a watt per candle, there was some way to go. During this same period, there were constant efforts to improve the filament, the obvious source of most of the inefficiencies and distortion in the bulb. A great many materials — fish, skin, bamboo, grass — had been tried out by a good many people in several different companies. By the turn of the century, Joseph Swan’s cellulose filament seemed best but not good enough. On the continent men were trying out metal. Von Bolton was working with tantalum at the Siemens Halske plant in Germany and Alexander Just and Franz Hanaman were experimenting with powdered Tungsten in Austria. So, at the start of the new century, there were, in the matter of the incandescent lamp, an imperfect solution, several very interesting technical problems, some promising investigations, a growing demand and — self-evidently — imposing rewards for the man or agency that would first come up with a bulb that burned brightly for a long time at low cost. — From Know-How to Nowhere
That was the state of affairs when, one day in 1900, three men from General Electric Headquarters in Schenectady went to see the former executive of their company Elihu Thomson. Thomson, who was once the engineering genius of what he and a partner built up into the Thomson-Houston Company — which eventually absorbed the Edison General Electric Company— was now on the faculty at MIT. He remained a consultant to his former company and was still influential in its affairs, but was spending most of his time conducting experiments to help flesh out the developing field of X-rays.
Thomson was a true lover of the process of finding things out and happened to make a fortune in the process. Ever since his childhood, he had been conducting his own chemical experiments in the attic of his house. He had a strong conviction that “the best contribution that could be made to God’s will was to study and understand nature and use it correctly (italics my own).”
The three men who came to see him were: Thomson’s former assistant who was now GE’s technical director, the manager of GE’s pattern department who had a strong enthusiasm for GE finding a way to make better lightbulbs before the Europeans, and a German-immigrant fellow named Steinmetz who GE had more or less been keeping around to do his own technical explorations because he was brilliant and off-the-charts curious. The three came to propose a novel idea: a laboratory that would carry out fundamental physical science research within GE.
Thomson loved the idea. An internal lab somewhat separate from day-to-day operations and commercial pressures where researchers were free to follow their curiosity was just what the company needed, Thomson thought. He strongly endorsed the idea, but he would not come on as director — which the three men had wanted. This was a delicate job that would require a man who understood all of the different forces at work. Thomson would have been perfect, but was caught up with his new life and research. Instead, he thought that Willis Whitney should come on as Director and assume the role of maintaining the balance of this new fundamental research lab that still needed to produce applied research but was also to be housed within a company that happened to have investors that did not care about scientific papers but cared a lot about profits.
Whitney accepted the challenge.
‘Are you still having fun?’
The new lab itself, located in a barn behind Steinmetz’s house, was an experiment in and of itself. Starting, initially, with just a barn and a bunch of problems related to the electrical field, Whitney (MIT undergrad, German Ph.D., MIT Professor of Physical Chemistry) set to work staffing the place with the right people and organizing it in such a way to allow these people to do their best work. Morison reflected on what would come of the nascent research operation:
There seems little doubt that in the next two decades much that was done in Schenectady in electrical engineering and some parts of physics was both better done and more interesting than what was being done in those fields in any American university.
Whitney routinely came into the lab and, as he looked into the state of things in different areas of work, would ask the researchers if they were having fun. Even when the course of research in some area was going quite hopelessly, he would ask it. Morison writes:
“Fun” was a favorite word of the director and those in the laboratory came in time to realize that the question meant, “Are you still working on that problem that no one else has the answer to?” which in turn meant, “Are you still engaged in the most exciting exercise there is in life?”
It’s no wonder why Thomson chose Willis Whitney for the job.
When people like Irving Langmuir started at the lab, Whitney would greet them and tell them to look around the lab for a few days. He wanted people like Langmuir to find out what in the varied work going on at the lab interested them most, and then go work on that strand of work they most enjoyed in any way they wished. So, Langmuir took a few days to look around, ask questions, and decide which area of work seemed most fun.
GE’s first (applied) bulb research czar
Langmuir (Columbia undergrad , German Ph.D., Chemistry Instructor at the Stevens Institute of Technology) came back to Whitney in a few days and said that the work related to the new bulb was what interested him most. At this time, in 1909, the interior walls of the bulb still had the tendency to blacken after only limited use. The laboratory investigations had previously shown that the blackening of the bulb decreased as the vacuum inside the bulbs increased. So, at that moment, much attention was going towards exploring ways to reach a more complete vacuum.
Langmuir was stepping into a line of research that was as old as the GE lab itself. While the bulb still had its problems, this line of research was considered a major success so far. One of the first courses of research Whitney oversaw at the lab was observing what would happen to many different kinds of materials under extreme heat (Read: sticking a bunch of interesting materials in a furnace and writing down what happened). Elting Morison writes of what happened after Whitney stuck a cellulose filament in the furnace for a while and it came out with metal-like properties:
In that form, he further discovered, it could convert electrical charges into light more efficiently and sustain the light for a longer time. What he had done increased the economy of the lamp’s performance, it was estimated, by 17 percent, or to 2.5 watts per candle. This gave the General Electric Company a significant competitive advantage in the market. So, at a cost of a million dollars, they built a new plant to manufacture these GEM lamps. So far, so good.
If the metallized carbonized cellulose filament was better, it stood to reason that some kind of actual metal filament would be an even further improvement, or, at least, it felt worth looking into. So, in 1905, Whitney asked William Coolidge to take charge in that exploration. Coolidge (MIT undergrad, German Ph.D., MIT Professor of Physical Chemistry) started by doing a course of experiments on the metal with the highest melting point: tungsten. It seemed like the clear place to start. He was not the first one to think of this idea. The Austrians, Just and Hanaman, had already been foiled by tungsten’s hardness. In its purest form tungsten is powder-like — which complicated the process. They were eventually able to work some magic and make a tungsten filament that could convert electrical charges into light efficiently, but the modifications they made to the tungsten to make this workable brought the shelf life of the bulb down to a commercially unacceptable level.
We won’t get into the details here, just know the final output of the Austrians’ process was known as sintered tungsten. Sintered tungsten didn’t work. Coolidge was going to attempt to make ductile tungsten — meaning you could draw the tungsten out into a thread-like shape — which he hoped would work. The investigation felt unpromising. Coolidge may have used the words “very unpromising” and “pretty nearly hopeless” to describe the endeavor. But, I guess in Whitney’s book, “pretty nearly hopeless” is just another way to describe fun, indefinite fun.
There were a lot of chemical properties about tungsten working against Coolidge’s efforts to make it ductile, but he did have a few things going for him. As Morison noted:
He knew a good deal about other metals and he knew, as any old time blacksmith did, that most metals increase in ductility as they approach their pure state and they are subjected to intensive “working” or pounding. Then, from his professional training, he knew a good deal about how to put order and system into an investigation.
(Read: do a series of exhaustive experiments until you learn to make the chemicals/materials behave the way you want them to behave.)
So, Coolidge set to work running experiment after experiment, trying to learn more about tungsten than anybody knew. He would heat the metal to varying temperatures and rolled, drew, and swaged samples of the metal at all temperatures (these are just different ways of working the metal that elicit different effects). He measured and recorded the effects of different permutations of temperatures and metal-working processes on the tungsten’s chemistry and structure. He then began mixing the tungsten with other substances and performing similar experiments to see what happened. In doing this constant experimentation, he began to build knowledge and intuition.
The steps taken were not (and could not have been) all planned out ahead of time, but the analysis of the steps was always carefully recorded and learned from. Morison continues:
Some way along in the series, he suspended tungsten powder in an amalgam of bismuth, cadmium, and mercury. He then passed the resulting substance through tiny dies — drawing it — and obtained a silvery pliable wire. At that time, he thought he had reached ductility and the search was over. But when a current was passed through this wire the mercury, cadmium, and bismuth distilled out, leaving, unfortunately, a nonductile tungsten. But it also proved to be tungsten in the purest state he had yet produced.
Fun.
Through this (exhaustive) commitment to his experimental process, Coolidge was able to find success. He eventually iterated his way to a workable process where a version of the more pure tungsten (from the above excerpt) was put through a specific combination of metal-working processes at a temperature that worked that produced rods of tungsten about 1 mm in diameter. These 1mm rods could then be drawn and re-drawn through rods of decreasing size until you were left with wires of tungsten .01 mm in diameter. When put in the vacuum-sealed bulb, electricity ran through the tungsten filaments and demonstrated an efficiency of 1 watt per candle — extending the life of a bulb up to 27x.
Within 5 years, 85% of all lamps would be made from tungsten. As the project went on, more and more research chemists and technical assistants grew to be involved in the wide-ranging steps and combinations involved in Coolidge’s experiments. But it worked. GE had the factories re-fit and deployed the new bulb. Coolidge moved on to other research.
And this was the area of the lab Irving Langmuir, after a few days of wandering around the lab, pointed out to Willis Whitney. He’d like to work over there.
A new kind of (fundamental) bulb research
Langmuir was about to put a completely different spin on this line of research. First of all, it should be noted, Langmuir did not even care about lightbulbs. Well, I guess that is not technically true. The bulb interested him because 1) he thought a metal like tungsten was cool because it could accept really high temperatures which opened up options to the scientist working with it and 2) these vacuum-sealed bulbs provided a pristine environment for controlled scientific investigations. But the bulb’s purpose, primarily interesting to most as an object that literally brought daylight inside on command, seemed less interesting to Langmuir than as a playground to do his science.
People in the lab had been studying how to improve the vacuum of the bulb since they assumed the imperfect vacuum was causing the bulb blackening. But Langmuir, mainly just wanting to learn some stuff, saw this as an opportunity to conduct a completely separate investigation.
If residual gases — imperfect vacua — produced a bad effect — blackening — here was a fine opportunity to study the effects produced by different gases introduced one by one into the bulb. What he wanted to do, he told Whitney, was simply to plot the interactions of various gases exposed at low pressures to very high temperatures in the filament. Nobody knew very much about this phenomena and he wanted to look into it simply “to satisfy [his] own curiosity.” — From Know-How to Nowhere
For three years, he carried on in this line of work. Not only were there, obviously, many gases and temperatures to try, but this initial line of work led off in many unforeseen directions that demanded investigations employing different methods altogether. In the end, what he learned did not help with the vacuum problem at all. He never even got started running experiments to improve the vacuum. By that metric, not forcing him to learn specifically about the bulb vacuum itself straight away was a mistake. And that is how many research operations, both now and back then, would have allocated Langmuir’s energies.
But luckily Langmuir worked under Willis Whitney. Whitney seemed to care that one worked doggedly in the process of discovery, but it didn’t have to solely consist of Cooldige-style, one-by-one tinkering and measuring-type investigations. Langmuir was mostly free to explore as he saw fit. But it should be noted that he was obligated to strike off on his explorations inspired by an existing course of research on a GE product with known limitations/bottlenecks that needed to be overcome.
Did Langmuir do anything to help seal the vacuum 5% better? No. But, thanks to Langmuir, we know that was a stupid problem. The following (chronologically ordered) ‘best hits’ of Langmuir’s filament work should give the reader an idea of how his desire for discovery manifested itself in fundamental work that was also at home in an industry R&D lab.
Langmuir found that during use the tungsten filament tended to evaporate — meaning the filament gave off a vapor just as water gives off steam. These tungsten vapor particles were finding their way onto the walls of the bulb. Those particles were what was causing the bulb blackening. Put simply: it was temperature, not an imperfect seal, causing the blackening problem.
Different gases markedly changed the rate of evaporation. One of the gases introduced during the experimentation was nitrogen which reduced the evaporation rate by 100-fold.
Building on this knowledge, tungsten filament bulbs filled with nitrogen were found to not blacken. But the addition of nitrogen caused the bulb to suffer heat loss and this made the electrical efficiency of the new bulb less efficient than the vacuum sealed, no nitrogen bulb.
But Langmuir inferred from prior fundamental work he had done that if one increased the diameter of the filament in the nitrogen-filled bulb, the amount of heat loss would be reduced. After some experimentation, he confirmed this intuition.
In trying to find other ways to reduce the heat loss problem, he also found that coiling the filament to a certain degree could achieve the same heat loss reduction as increasing the filament diameter.
Putting all this together in one bulb, he found that if you used an inert gas instead of a vacuum to reduce bulb blackening— nitrogen in the beginning but later switching to argon — and coiled the tungsten filament one could make a bulb that required only one half of one watt per candle and lasted three times longer than any other bulb.
Langmuir’s discoveries were often born out of his investigations of anomalies he encountered in the laboratory. Many of his publications on these laboratory encountered phenomena were quite mathematical in nature and aimed at outlining/modeling the fundamental laws and properties of the phenomena which he was observing. In this way, Langmuir’s work was markedly differentiated from Coolidge’s (If you’re curious, take a look at the following papers by Langmuir and Coolidge and you’ll see just how much math and theoretical modeling Langmuir used and how little Coolidge used). To give you an idea of the Irving Langmuir Production Function, below is an excerpt from a letter he wrote to Scientific Monthly talking about his experiences with fundamental research in the GE Lab:
In working with nitrogen, I found that the nitrogen had a peculiar tendency to disappear, and was able to prove (combining high-level theory and experimentation) that this was due to a chemical reaction by which each tungsten atom which evaporated from the filament combined with nitrogen to form a compound WN₂. In order to study this reaction I needed to determine the rate of loss of weight of tungsten filaments at different temperatures. I found that this loss of weight was due to evaporation, and not, as had been previously considered, to an effect of electric discharges. When working with nitrogen at atmospheric pressure, the rate of evaporation was decreased about a hundredfold because of the return of the tungsten atoms to the filament after they collided with nitrogen molecules in the gas.
I had noticed that with nitrogen at atmospheric pressure it was possible to maintain a filament at a temperature close to the melting point for a far longer time than if the filament were in a vacuum.
The applicability and profitability of his findings was not a one-off either. Once he passed this light bulb project off to the later development and engineering stages, he set his sights on studying electric discharges in lamps. As he had learned in carrying out this previous course of research, only a few milliamperes of current would flow across the space between one end of the filament and the other even with a current higher than 100 volts. Langmuir remarked in his letter to Scientific Monthly:
This fact seemed very peculiar to me, for the work of Richardson and others had indicated that at temperatures as high as those used in the tungsten-filament lamp, currents of many amperes should flow across the space. In other words, according to the then-accepted theory of the electron emission from hot filaments, a serious difficulty should have been encountered in the construction of tungsten-filament lamps. The fact that we did not meet any such difficulty therefore seemed to me a peculiar fact that should be investigated.
With only a few days of work on the problem, Langmuir discovered what would be known as the space-charge effect. In short, Langmuir writes:
The electrons that escape from the hot filament require a definite time to cross the space to the positively charged end of the filament, and while these electrons are crossing the space they are repelling those that are leaving the filament behind them, therefore there is a definite limit to the current that can flow with a given impressed voltage.
Coolidge, who was previously working on the light bulb project, was now working on a course of research studying the use of tungsten electrodes for X-ray tubes. He was able to deploy Langmuir’s newfound understanding of high vacuum discharge to construct an entirely new kind of X-ray tube with a stronger vacuum and a hot tungsten filament as cathode.
The understanding of the space-charge effect also helped the folks in the lab understand why a device called an Audion, utilized in detecting and amplifying radio signals, inexplicably required a poor vacuum seal (which let in some gas) to work properly. This new understanding allowed the lab to construct an Audion so powerful that it could now be used for radio telephony and broadcasting.
The relationship between Langmuir and the lab was not only symbiotic in that the lab gave Langmuir money and in return he gave them research. The lab gave Langmuir a seemingly endless supply of problems and anomalies to consider in his research. The following (additional) list of Langmuir’s subsequent research in the lab show both how Langmuir continued to find fundamental research ideas in the applied setting and how his research continued to bear fruit for GE. (the reader can skip these bullets if they wish)
Building on Langmuir’s work on heat loss from tungsten filaments, “Professor R. W. Wood showed that when an electric discharge is passed through hydrogen at low pressures atomic hydrogen is formed, and that this can recombine on the surface of metal wires and cause them to be heated.” Langmuir, seeing this and fitting it together with his past experiences in the GE Lab, led him to the belief that using hydrogen at atmospheric pressure with a high current could be even more effective. This led to a very useful hydrogen welding process that enabled the construction of sealed-in electric refrigerator units.
He conducted some studies of the peculiar way in which tungsten vapor condensed (later also using mercury and cadmium) on glass surfaces and came to the conclusion that some of the accepted ideas about condensation were incorrect. The prior theories likened these particles’ behavior to reflecting off surfaces at certain temperatures. He showed that the phenomena “could be better explained by assuming that the molecules or atoms on striking the surface always condensed, and that then, after a certain time interval depending on the temperature, they would evaporate off.” (This was the beginning of a line of work in surface chemistry that is credited with winning him the Nobel Prize.)
Langmuir then saw that he could use this newfound knowledge to understand and overcome limitations in the diffusion pump and then constructed the improved model, the condensation pump, which immediately increased the speed of the mercury-vapor pump from 80 cc to about 4,000 cc per second.
The tungsten filament and gases work led Langmuir to recognize the importance of a single layers of atoms on a surface in determining the properties of that surface. He carried out investigations of these single layer of atoms on solids, liquids, and gases. This work initially led Langmuir and his team to more precisely measure the dimensions of molecules of many substances as well as measure the absolute values of wavelengths and X-rays better than was previously possible.
Coolidge and Langmuir both did phenomenal research. But for Coolidge, it was more the system of experiments and measurements themselves driving his work. Many years later, Coolidge himself reflected on what drove his success in the filament project:
I must say…that we were guided in the main by experiment itself rather than by metallurgical knowledge.
Contrary to Coolidge, Langmuir, when reflecting on the development of the gas-filled bulb remarked that it was not the product of engineering but of “lots of different lines of pure scientific work.” Morison elucidates this contrast further:
Where before men had proceeded doggedly and step by step to improve performance by improving the existing given conditions — from horsehair to tungsten, from imperfect to less imperfect vacua — he [Langmuir] had gone by a different path until he arrived at new ideas that could then be translated into a bulb of a different design based on a different concept. No lamp existing in 1911, he said, would have gained in efficiency or life expectancy by the addition of nitrogen or argon. What had been done, he concluded, was to derive a “new kind of lamp from new scientific principles.”
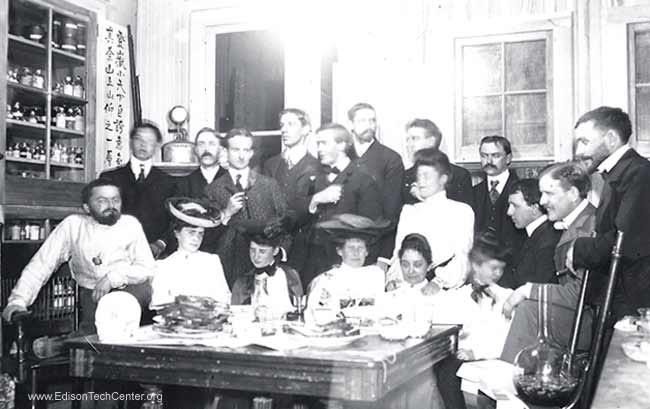
When applications lead to theory
There are many takeaways from this fact pattern. Langmuir’s own essay in Scientific Monthly, in which he describes much of this work, was primarily attempting to argue that some brand of fundamental research can have a productive place in industry. And, while I also believe that, that is not exactly a contrarian opinion (at all) in the progress community. What I’d like to talk about is not Langmuir’s impact on the GE Laboratory and the company as a whole, but, rather, the GE Lab’s impact on Langmuir’s work.
In the Langmuir Production Function, observing what was going on in the lab was a critical component. What can and can’t the applied researchers/engineers do and why? What are they currently doing that the literature actually says is impossible? Is there anything that should be possible that they seemingly can’t do? These were the types of questions that defined Langmuir’s career (and many like him).
In a developed world that is not exactly beset by scarcity and hardship anymore, it is hard to come up with the best areas to explore out of thin air. Pain points are not often obvious. Fundamental researchers can benefit massively from going to a lab mostly dedicated to making practical improvements to things like light bulbs and pumps and observing/asking questions. It is, frankly, odd that we normalized a system in which so many of our fundamental STEM researchers are allowed to grow so disjoint from the applied aspects of their field in the first place.
And if you still believe that Langmuir would have found problem areas as good or better if he was off on his own, that very may well be. But…Irving Langmuir himself would probably strongly disagree with you. Langmuir did, on the one hand, agree that a level of freedom in this fundamental research done within corporate R&D labs was vital. He wrote:
Such research can not usually be directed toward definite goals, for it involves un- known factors. Success in such research, if attained, is often reached by wholly unexpected methods, and the problem which is finally solved is not the problem which is foreseen.
And that is the kind of stuff this generation of scientists, who did a relatively large amount of work in applied settings, wrote down and advocated for that people tend to remember. But what is forgotten is how many of these individuals also explicitly laid out that they imagined this free-flowing, fundamental research being much more tied in with applied research than it tends to be today. Langmuir immediately followed the quote above with:
As this laboratory developed it was soon recognized that it was not practicable nor desirable that such a laboratory should be engaged wholly in fundamental scientific research. It was found that at least 75 per cent of the laboratory must be devoted to the development of the practical applications. It is stimulating to the men engaged in fundamental science to be in contact with those primarily interested in the practical applications. It is also important that the engineers in the organization should be in close contact with those having the broader scientific outlook.
Langmuir may have been able to answer a large portion of his experimental questions on his own within a university, but he would have known to ask very few of them. Small anomalies and curiosities came up in the lab’s work. He looked into them. Sometimes he found clean answers, sometimes it was even more questions, and others it was even that the academics had been getting something entirely wrong.
He was not pulling genius out of thin air. That kind of genius in science is rare, and I rarely come across individuals like this in my research. And, as a note, the product ideas were also not exactly being dreamed up ahead of time by some visionary. Langmuir reflected on the space-charge effect work, one of many areas in which these small and seemingly directionless investigations based on a curiosity from the lab’s work led to an application, noting:
There was no one in the General Electric Company who was conscious of the need of a high-powered electron amplifier. However, once we were led to the construction of such a device we could immediately think of an enormous number of applications for it.
A grand roadmap-like plan for a lab or individual is unreasonable. Pulling brand new thoughts or unseen contradictions that exist in the field out of the sky is rare. But running into problems while doing applied research and engineering development work is as common as finding a bug in a function you are trying to write. If it doesn’t happen, you’re surprised.
Pulling the vast majority of fundamental scientific researchers out of this setting was, in my estimation, a big mistake. Morison, in writing the chapters of this book strove to remind readers just how reciprocal the processes of building and discovery are, particularly when the applications are quite scientific in nature. He continues, stating that the history of this reciprocity shows:
That one can begin at either end of the process and move towards the other end—from the tungsten filament out to some “new scientific principles” or from those principles back down to the argon bulb. It suggests also the far more powerful results obtained when the connections in this reciprocal process are brought within the enforced intimacy and continuity of an organized system, that is, when the whole process is institutionalized.
I’d imagine if one were to go back in time and tell Morison that a relatively unproductive era of American innovation would immediately follow the breakdown of this reciprocal system, he’d have not exactly been shocked. Without this process, effectively organizing the process and translation of discovery is quite difficult.
(Another) Fun idea for a new science org to pilot
I believe we should find ways, wherever possible, to reintroduce fundamental researchers to the processes and applied settings to which they are meant to contribute. The better portion of a great generation of researchers who we idolize, like Langmuir, have the fingerprints of their applied experiences all over their more fundamental work.
What this process would look like would vary from field to field and based on the imaginations and ambitions of the funders and researchers involved. But the concept is quite straightforward.
Finding scientific problems and anomalies to think about can be particularly challenging. Challenging grad students and young researchers to find and fill holes in the academic literature of some field is one way to organize researchers’ energies as they attempt to contribute to knowledge/societal good. It’s not the worst heuristic and there will always be a place for some of this “hole-filling” in the literature. However, I think it is a mistake to let this continue to overpower application-based and application-inspired explorations to the extreme degree that it has.
In my Progress Studies History of Early MIT series, particularly Part 2, I outlined how this kind of symbiotic applied and basic research ecosystem can (and has been) fostered in the university setting. In this piece, I illustrated a piece of history that can serve as inspiration for any new science org looking to prove the merits of this mutually beneficial model in the setting of a stand-alone research lab or a research lab affiliated with a larger organization.
These labs really were a good bit of fun. And many talented people who read this Substack reach out to me express strong interest in working in research environments like the one I describe at the GE Laboratory. Any academic area with 1) clear ties to some real-world application (whether real science or social science), 2) that some funder is excited about, and 3) where individual experiments/analyses can be run relatively cheaply would be an ideal place to start.
If anyone is interested in discussing what this would look like in practice, please reach out to me on Twitter. I’d love to help.
Once again, thanks for reading the FreakTakes Substack :) Please reach out on Twitter if you’d like to discuss anything from the piece.
Citation:
Gilliam, Eric. “Irving Langmuir, the General Electric Research Laboratory, and when applications lead to theory,” FreakTakes Substack. 2022. https://freaktakes.substack.com/p/irving-langmuir-the-general-electric
Morison was a founding member of MIT’s STS (Science, Technology, and Society) Department which Gerald Holton, whose work was covered in this Substack’s (by far) most well-known piece, was also instrumental in helping establish. I’ve heard several historians say that neither of these two men is, strictly speaking, a real science historian because they don’t use the strict methodology of the field.
And, frankly, I would believe that. Reading strictly written work from historians is useful for the field but often quite boring for people like you and me. Morison’s work, in contrast, is a pleasure to read. So, while Morison and Holton might not be real historians, they’re absolutely godfathers of progress studies. If you like this piece, I encourage you to get a copy of the book here. And if you can’t because it’s out of stock, just tweet at Tammy Winter at Stripe Press and she might get the book back in print for us if enough of you ask! It has worked before…