A cortical-hippocampal-cortical loop of information processing during memory consolidation
- PMID: 27941790
- PMCID: PMC5783826
- DOI: 10.1038/nn.4457
A cortical-hippocampal-cortical loop of information processing during memory consolidation
Abstract
Hippocampal replay during sharp-wave ripple events (SWRs) is thought to drive memory consolidation in hippocampal and cortical circuits. Changes in neocortical activity can precede SWR events, but whether and how these changes influence the content of replay remains unknown. Here we show that during sleep there is a rapid cortical-hippocampal-cortical loop of information flow around the times of SWRs. We recorded neural activity in auditory cortex (AC) and hippocampus of rats as they learned a sound-guided task and during sleep. We found that patterned activation in AC precedes and predicts the subsequent content of hippocampal activity during SWRs, while hippocampal patterns during SWRs predict subsequent AC activity. Delivering sounds during sleep biased AC activity patterns, and sound-biased AC patterns predicted subsequent hippocampal activity. These findings suggest that activation of specific cortical representations during sleep influences the identity of the memories that are consolidated into long-term stores.
Conflict of interest statement
The authors declare no competing financial interests.
Figures
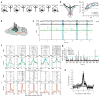
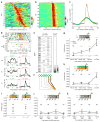
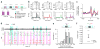
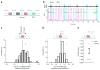
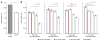
Similar articles
-
Hippocampal-Prefrontal Reactivation during Learning Is Stronger in Awake Compared with Sleep States.J Neurosci. 2017 Dec 6;37(49):11789-11805. doi: 10.1523/JNEUROSCI.2291-17.2017. Epub 2017 Oct 31. J Neurosci. 2017. PMID: 29089440 Free PMC article.
-
Hippocampal ripples as a mode of communication with cortical and subcortical areas.Hippocampus. 2020 Jan;30(1):39-49. doi: 10.1002/hipo.22997. Epub 2018 Nov 13. Hippocampus. 2020. PMID: 30069976 Review.
-
Exposure to sounds during sleep impairs hippocampal sharp wave ripples and memory consolidation.bioRxiv [Preprint]. 2023 Nov 22:2023.11.22.568283. doi: 10.1101/2023.11.22.568283. bioRxiv. 2023. PMID: 38045371 Free PMC article. Preprint.
-
Coordination of Human Hippocampal Sharpwave Ripples during NREM Sleep with Cortical Theta Bursts, Spindles, Downstates, and Upstates.J Neurosci. 2019 Oct 30;39(44):8744-8761. doi: 10.1523/JNEUROSCI.2857-18.2019. Epub 2019 Sep 18. J Neurosci. 2019. PMID: 31533977 Free PMC article.
-
The hippocampal sharp wave-ripple in memory retrieval for immediate use and consolidation.Nat Rev Neurosci. 2018 Dec;19(12):744-757. doi: 10.1038/s41583-018-0077-1. Nat Rev Neurosci. 2018. PMID: 30356103 Free PMC article. Review.
Cited by
-
Emerging many-to-one weighted mapping in hippocampus-amygdala network underlies memory formation.Nat Commun. 2024 Oct 26;15(1):9248. doi: 10.1038/s41467-024-53665-9. Nat Commun. 2024. PMID: 39461946 Free PMC article.
-
The evolving view of replay and its functions in wake and sleep.Sleep Adv. 2021 Jan 30;1(1):zpab002. doi: 10.1093/sleepadvances/zpab002. eCollection 2020. Sleep Adv. 2021. PMID: 33644760 Free PMC article. Review.
-
Single-trial cross-area neural population dynamics during long-term skill learning.Nat Commun. 2020 Aug 13;11(1):4057. doi: 10.1038/s41467-020-17902-1. Nat Commun. 2020. PMID: 32792523 Free PMC article.
-
Real-time classification of experience-related ensemble spiking patterns for closed-loop applications.Elife. 2018 Oct 30;7:e36275. doi: 10.7554/eLife.36275. Elife. 2018. PMID: 30373716 Free PMC article.
-
The hearing hippocampus.Prog Neurobiol. 2022 Nov;218:102326. doi: 10.1016/j.pneurobio.2022.102326. Epub 2022 Jul 21. Prog Neurobiol. 2022. PMID: 35870677 Free PMC article. Review.
References
-
- Frankland PW, Bontempi B. The organization of recent and remote memories. Nat Rev Neurosci. 2005;6:119–130. - PubMed
-
- Squire LR, Alvarez P. Retrograde amnesia and memory consolidation: a neurobiological perspective. Curr Opin Neurobiol. 1995;5:169–177. - PubMed
-
- Eichenbaum H. A cortical-hippocampal system for declarative memory. Nat Rev Neurosci. 2000;1:41–50. - PubMed
-
- Buzsáki G. The hippocampo-neocortical dialogue. Cereb Cortex. 1996;6:81–92. - PubMed
Publication types
MeSH terms
Grants and funding
LinkOut - more resources
Full Text Sources
Other Literature Sources