Whole-brain activity mapping onto a zebrafish brain atlas
- PMID: 26778924
- PMCID: PMC4710481
- DOI: 10.1038/nmeth.3581
Whole-brain activity mapping onto a zebrafish brain atlas
Abstract
In order to localize the neural circuits involved in generating behaviors, it is necessary to assign activity onto anatomical maps of the nervous system. Using brain registration across hundreds of larval zebrafish, we have built an expandable open-source atlas containing molecular labels and definitions of anatomical regions, the Z-Brain. Using this platform and immunohistochemical detection of phosphorylated extracellular signal–regulated kinase (ERK) as a readout of neural activity, we have developed a system to create and contextualize whole-brain maps of stimulus- and behavior-dependent neural activity. This mitogen-activated protein kinase (MAP)-mapping assay is technically simple, and data analysis is completely automated. Because MAP-mapping is performed on freely swimming fish, it is applicable to studies of nearly any stimulus or behavior. Here we demonstrate our high-throughput approach using pharmacological, visual and noxious stimuli, as well as hunting and feeding. The resultant maps outline hundreds of areas associated with behaviors.
Figures
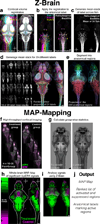
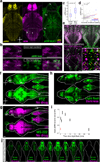
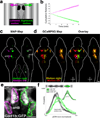
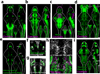
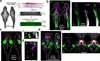
Similar articles
-
A dynamic and expandable digital 3D-atlas maker for monitoring the temporal changes in tissue growth during hindbrain morphogenesis.Elife. 2022 Sep 28;11:e78300. doi: 10.7554/eLife.78300. Elife. 2022. PMID: 36169400 Free PMC article.
-
A Brain-wide Circuit Model of Heat-Evoked Swimming Behavior in Larval Zebrafish.Neuron. 2018 May 16;98(4):817-831.e6. doi: 10.1016/j.neuron.2018.04.013. Epub 2018 May 3. Neuron. 2018. PMID: 29731253 Free PMC article.
-
Seeing the whole picture: A comprehensive imaging approach to functional mapping of circuits in behaving zebrafish.Neuroscience. 2015 Jun 18;296:26-38. doi: 10.1016/j.neuroscience.2014.11.046. Epub 2014 Nov 27. Neuroscience. 2015. PMID: 25433239 Review.
-
Brain-wide circuit interrogation at the cellular level guided by online analysis of neuronal function.Nat Methods. 2018 Dec;15(12):1117-1125. doi: 10.1038/s41592-018-0221-x. Epub 2018 Nov 30. Nat Methods. 2018. PMID: 30504888
-
Two-photon imaging of neural population activity in zebrafish.Methods. 2013 Aug 15;62(3):255-67. doi: 10.1016/j.ymeth.2013.05.016. Epub 2013 May 31. Methods. 2013. PMID: 23727462 Review.
Cited by
-
Glial cell derived pathway directs regenerating optic nerve axons toward the CNS midline.bioRxiv [Preprint]. 2024 Oct 18:2024.10.15.618346. doi: 10.1101/2024.10.15.618346. bioRxiv. 2024. PMID: 39464127 Free PMC article. Preprint.
-
Two-photon all-optical neurophysiology for the dissection of larval zebrafish brain functional and effective connectivity.Commun Biol. 2024 Oct 4;7(1):1261. doi: 10.1038/s42003-024-06731-3. Commun Biol. 2024. PMID: 39367042 Free PMC article.
-
Brain-Body Control of Glucose Homeostasis-Insights From Model Organisms.Front Endocrinol (Lausanne). 2021 Mar 31;12:662769. doi: 10.3389/fendo.2021.662769. eCollection 2021. Front Endocrinol (Lausanne). 2021. PMID: 33868184 Free PMC article. Review.
-
Hypothalamic Dopamine Neurons Control Sensorimotor Behavior by Modulating Brainstem Premotor Nuclei in Zebrafish.Curr Biol. 2020 Dec 7;30(23):4606-4618.e4. doi: 10.1016/j.cub.2020.09.002. Epub 2020 Oct 1. Curr Biol. 2020. PMID: 33007241 Free PMC article.
-
Evaluation of Neural Regulation and Microglial Responses to Brain Injury in Larval Zebrafish Exposed to Perfluorooctane Sulfonate.Environ Health Perspect. 2023 Nov;131(11):117008. doi: 10.1289/EHP12861. Epub 2023 Nov 15. Environ Health Perspect. 2023. PMID: 37966802 Free PMC article.
References
-
- Fero K, Yokogawa T, Burgess HA. Neuromethods. Vol. 52. Humana Press; 2010. pp. 249–291.
-
- Ahrens MB, Orger MB, Robson DN, Li JM, Keller PJ. Whole-brain functional imaging at cellular resolution using light-sheet microscopy. Nat Meth. 2013;10:413–420. - PubMed
-
- Fosque BF, et al. Labeling of active neural circuits in vivo with designed calcium integrators. Science. 2015;347:755–760. - PubMed
-
- Nava SS, An S, Hamil T. Visual detection of UV cues by adult zebrafish (Danio rerio) Journal of Vision. 2011;11:2–2. - PubMed
Methods-only references
-
- Scott EK, et al. Targeting neural circuitry in zebrafish using GAL4 enhancer trapping. Nat Meth. 2007 - PubMed
-
- Wen L, et al. Visualization of monoaminergic neurons and neurotoxicity of MPTP in live transgenic zebrafish. Developmental Biology. 2008;314:84–92. - PubMed
-
- McLean DL, Fan J, Higashijima S-I, Hale ME, Fetcho JR. A topographic map of recruitment in spinal cord. Nature. 2007;446:71–75. - PubMed
Publication types
MeSH terms
Substances
Grants and funding
LinkOut - more resources
Full Text Sources
Other Literature Sources
Molecular Biology Databases
Research Materials
Miscellaneous