A comprehensive review of research methods used for studying the binding of a protein receptor and its ligand.
Physiological processes are regulated by molecular mechanisms involving protein-protein and protein receptor-ligand interactions. Quantification of ligand binding to specific receptors is a key concept of both theoretical studies and drug development research. The main aspects of ligand-receptor binding interactions include binding affinity and kinetics, conformations of targets, binding thermodynamics and ligand efficiency. Every step of ligand-target interactions can be analyzed by different ligand-binding assays. Ligand-binding assays include labeled, label-free, structure-based, thermodynamic, and whole cell ligand-binding assays (Table 1).
Group of assays | Assay | Mechanism | Advantages | Disadvantages |
---|---|---|---|---|
Labeled ligand-binding assays | Fluorescent ligand binding assays | Fluorescent labeled ligand binding assay is applied to detect its binding to a target. | These assays have a broad spectrum of wavelengths, therefore it can apply multiple colors. | The assays are susceptible to different fluorescence interferences. Also, the labeling of a ligand, can lead to undesirable alterations in the binding characteristics of the ligand. In addition, it is difficult to obtain good fluorescent ligands for many receptors. |
Radioligand binding assays | Radioactively labeled ligand binding assay is applied to detect its binding to a target. | The most popular assay for membrane-bound targets. Good robustness, precise determination of receptor density and distribution, ligand binding sites and affinity. | High cost and hazards of handling high levels of radioactivity. | |
Bioluminescent binding assays using nanoluciferase | Bioluminescent reporter nanoluciferase (NanoLuc) is used to study interactions of protein hormones with their receptors. | NanoLuc-based assays have several advantages: high sensitivity, high stability and reproducibility. | Since NanoLuc reporter is larger than radioisotopes, it may potentially influence the receptor binding. | |
Label-free ligand binding assays | Surface plasmon resonance (SPR) | SPR applies light-excited surface plasmon polaritons to track the binding of ligands to the receptors bound to a gold surface. | SPR is the most popular label-free ligand binding assay. This assay can be used to determine binding kinetics. | This assay has low sensitivity to binding-induced conformational change. |
Plasmon-waveguide resonance (PWR) | PWR applies a continuous polarized wave laser exciting electromagnetic waves in a resonator made of a thin silver film with a layer of SiO2 and a glass prism. Ligand binding changes amplitude, position and width of reflected lights. | PWR examines anisotropic optical contents of receptor-ligand complexes, thus differentiating mass density changes from conformational changes. | PWR has lower sensitivity than SPR with regard to refractive index, thickness and mass parameters. | |
SPR imaging for affinity-based biosensors | This assay measures the binding kinetics and is related to intensity modulation and analyzes the reflectivity of monochromatic incident p-polarized light detected at a fixed angle. | This assay can be used to monitor binding events in real time. | This assay characterizes different compounds for a specific target, it still provides low throughput. | |
Nanofluidic Fluorescence Microscopy (NFM) | NFM combines nanofluidic-based biosensors and fluorescence microscopy to analyze the protein binding kinetics. | Protein binding kinetics can be analyzed in one single experiment. The time of analysis by NFM is shorter compared to other microfluidic assays. Statistical errors are reduced and there are no limitations on the size of the analyzed molecules. | Limitations of fluorescence microscopy. | |
Whispering gallery microresonator (WGM) | WGM is based on wave sensing binding of molecules to the surface of the cavity induces changes of the resonant wavelength changes. The resonant changes of light permit multiple analyses of molecules. | WGM is a highly sensitive assay for molecule detection. | The possibility to study drug-target interactions under non-equilibrium conditions using this assay is still unclear. | |
Resonant waveguide grating (RWG) | RWG uses a nanograting to couple light into the waveguide via diffraction. The light illuminates the biosensors in microplate at a nominally normal incident angle. The drug binding of the immobilized receptors results in a shift in the resonant wavelength. | RWG assay is effective for affinity screening. | This assay is not applicable to study cells due to the large size of cells and limited penetration ability of an evanescent wave. | |
Biolayer Interferometry Biosensor (BIB) | This assay uses a spectrometer to detect interference patterns formed by light reflected from an optical layer and a biolayer containing proteins of interest. | Effective assay to study binding kinetics. | This assay has been validated mostly for small molecule detection. | |
Structure-based ligand binding assays | Nuclear magnetic resonance (NMR) | NMR analyzes the magnetic characteristics of certain atomic nuclei, which absorb electromagnetic radiation in the magnetic field. | NMR can be applied to analyze the structure of proteins and the molecular details of protein-ligand interactions and to assist structure-based drug design. Besides, NMR can be used for any class of compounds, for almost all soluble proteins, natively unstructured proteins or membrane proteins. | The high cost of the assay, time-consuming, requires a long time to analyze obtained spectra. The resolving power of NMR is lower than X-ray crystallography. |
X-ray crystallography | This assay measures the changes of the diffracted X-ray beams in order to produce a three-dimensional image of electron density within the molecule of interest. Obtained image of electron density is used to determine the structure of the molecules. | The solvent effect can be examined by X-ray crystallography. The whole 3D structure can be obtained by the systematic analysis of a crystallized material. | Solutions and behavior of molecules in solution cannot be examined. Direct determination of secondary structures including domain movements cannot be performed. | |
Thermodynamic binding assays | Thermal denaturation assays (TDA) | TDA detect the thermal denaturation of proteins by differential scanning fluorimetry, which applies a prob fluorophore to monitor thermal denaturation process of proteins in the presence and ligands. | These methods have found applications in chemical profiling of different protein families, identifying novel ligands, and investigating the stability of proteins in buffers in solution | These methods may not be suitable for analysis of very small proteins that do not aggregate upon denaturation. |
Isothermal titration calorimetry (ITC) | ITC measures the binding enthalpy variation by sensing the heat caused by the binding reaction | This method is useful for detecting highly potent and entropy-driven ligands, compared to others. | This method shows low throughput and sensitivity, and requires large sample volumes. | |
Whole cell ligand-binding assays | Surface acoustic wave (SAW) biosensor | The surface of the biosensor is excited to oscillate with a high frequency. The phase of the generated acoustic wave is shifted upon mass changes. Conformational characteristics are indicated by a change in amplitude. | Using this assay, the sequential binding of several distinct ligands, targeting different cell surface molecules, to the whole cells can be detected directly in real time. | SAW biosensors for analysis of small molecules using living cells have not developed. |
RWG biosensor | RWG biosensor can transform drug-receptor interactions in cells into cell phenotypic signatures named dynamic mass redistribution. | This assay measures the consequence of binding, and the stimulus-response interpretation of the initial interaction of drug and receptor. Also, RWG can analyze the functional consequences of ligand binding and dissociation. | RWG biosensor may not be used to detect ligand binding directly. |
Fluorescence has a wide spectrum of wavelengths, therefore multiple colors can be applied for the detection of a specific target. Multiple techniques such as fluorescence anisotropy, fluorescence correlation spectroscopy, time-resolved fluorescence, fluorescence polarization, fluorescence and bioluminescence resonance energy transfer are used to measure fluorescence intensity. All of them have been applied in ligand binding assays. Fluorescence anisotropy can be applied to measure unequal intensities of the light emitted from a fluorophore along different axes of polarization [4]. An example is the recent study on the ligand binding dynamics of G protein-coupled receptors (GPCRs) [5]. Fluorescence correlation spectroscopy analyzes fluctuations of the fluorescence intensity, measuring the average number of fluorescent particles and diffusion time, detecting the passage of the particles through space [6]. Time-resolved fluorescence applies convolution integral to measure the lifetime from fluorescence decay after excitation [7]. Fluorescence polarization method detects the difference between the polarization of the polarized light used to excite the fluorophore and light emitted from a fluorophore [8].
Resonance energy transfer methods apply labels which are attached to reactive amino acid side chains. These labels include fluorescent proteins and light-emitting enzymes. Fluorescence and bioluminescence resonance energy transfer (FRET and BRET) methods are used to monitor the distances between two labels. These methods analyze conformational changes of single protein molecules or protein-protein interactions. Furthermore, FRET method detects the non-radiative transfer of energy from one donor chromophore to an acceptor chromophore (Figure 1) [1]. The method was recently used to decipher the interactions between the estrogen alpha receptor and its co-activator [9], to screen GCPR ligand libraries [10] and to detect receptor-ligand binding in situ [11], leading the way to visualizing such interaction in their native environment. Zhao LH et al detected FRET to measure the binding kinetics between GFP-tagged parathyroid hormone receptor-1 and tetramethylrhodamine-labeled ligand in live HEK-293 cells [12].
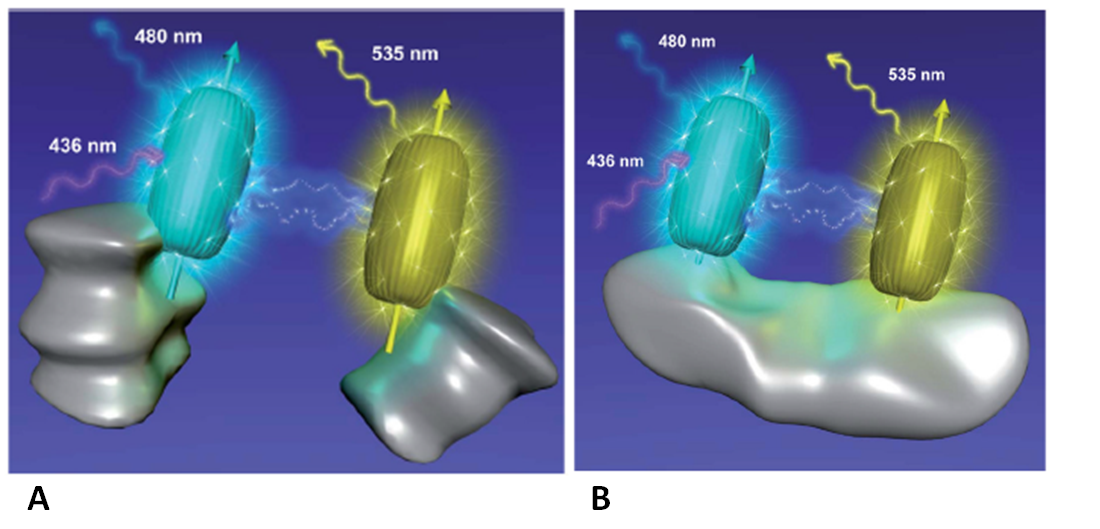
Recent studies applied a combination of multi-channel fluorescence imaging with microarrays to study molecular interactions. Protein microarrays such as kinase and G protein-coupled receptor (GPCR) arrays have been designed and applied to analyze ligand binding [13]. Analysis of protein kinase A and leukocyte-specific protein tyrosine kinase with 80 kinase inhibitor library confirmed previously identified inhibitors for these two kinases [14].
Multiple fluorescent ligands have been developed for the studies of their specific receptors. Among the most recent are ligands for the GPCR receptors [15], bradykinin B1 receptor [16], sigma-1 receptor [17], μ-opioid receptor [18], the 5-HT3 receptor [19], and the oxytocin receptor [20].
Recent improvements in the technique allowed for the monitoring of protein kinetics in real time (e.g., by using nanofluidic fluorescence microscopy [21] ), and the quantitative analysis of protein-ligand interactions [22].
A radioactively labeled ligand is applied in this assay to detect its binding to a target. Radioligand binding assays are mainly used for membrane-bound molecules, such as G protein-coupled receptors like angiotensin II type 1 receptor [23] or apelin receptor [24]. Radioligand binding assays can determine the type, anatomical distribution and density of the receptors and ligand affinity and binding sites [25]. There are three experimental types of radioligand binding assays: saturation assay, competitive assay and kinetic assay [26]. Saturation assays analyze the equilibrium binding of radioactively labeled ligand to the receptor, using a fixed receptor level and increasing concentrations of the ligand. The assay measures the tissue / cell-specific affinity and the density of the analyzed receptor. A competitive assay investigates equilibrium binding at a fixed concentration of radioligand and in the presence of different concentrations of an unlabeled competitor, for example, [12, 23, 24, 27]. Binding data analysis provides the affinity of the receptor for the competitor molecule. Kinetic assays are used to determine receptor / ligand pair specific dissociation and association constants. Multiple receptor specific radioligand binding assays protocols have been developed [28]. Zhao LH et al measured the binding affinity of wild-type and mutant parathyroid hormone receptor-1 against its ligand in HEK-293T cells through radioiodine-labeled parathyroid hormone in the presence of varying concentrations of unlabelled ligand [12]. The binding can be measured through a scintillation proximity assay [27, 29] or a polyethylene glycol precipitation assay [29].
Nanoluciferase (NanoLuc)-based methods have several important advantages, including high sensitivity and reproducibility, and were applied to the BRET analysis [30], in vivo imaging [31], and membrane internalization assessment [32]. Recently, NanoLuc has been effectively used in binding assays for various protein hormones and their receptors. Several studies reported applications of NanoLuc-based assays for the detection of relaxin [33], ghrelin [34] and erythropoietin [35]. In addition, NanoLuc was recently used in the development of the BRET-based assessment of the G Protein-Coupled Receptors (GPCR) ligand binding [15]. Both chemical conjugation and genetic fusion may be applied for the development of bioluminescent binding assays. With regard to the chemical conjugation technique, the selected protein hormones are specifically conjugated with a NanoLuc reporter. The NanoLuc-bound proteins can be isolated by chromatography and analyzed by bioluminescence techniques.In contrast to chemical conjugation, the genetic approach applies fusion of the bioluminescent reporter with a chosen protein domain, followed by the overexpression of the generated complex in the bacterial or mammalian cells. In particular, genetic fusion was reported to be effective for the analysis of interactions of erythropoietin with its membrane receptor [35].
Selenium labeling of peptide ligands, coupled with inductively coupled plasma mass spectrometry, has been evaluated for measuring the receptor-ligand interaction [36].
Surface plasmon resonance is based on the surface plasmon polaritons (SPP) which are electromagnetic waves generated when light interacts with surface charges in the gold surface (Figure 2). The main application of SPR is to analyze the binding of the ligands to the receptors linked to a gold surface. Therefore, SPR is used to study binding kinetics [37]. The analyzed interactions between a drug and its target include the formation of a ligand-receptor complex and dissociation of this complex followed by the ligand removal. SPR is applied to follow the association and dissociation mechanisms in real time by creating a sensorgram which detects the changes in wavelength. Importantly, SPR is a quantitative method, since these changes are related to the number of bound ligand molecules. However, the sensitivity of SPR is low in the detection of binding-induced conformational change [38]. SPR assay can be used for different soluble proteins including kinases and proteases, as well as for membrane protein [39]. In particular, SPR assay has recently been used to analyze the binding of extracelluar domains of membrane proteins [40], the interaction of orthosteric and allosteric ligands with chemokine receptor, CCR5, lipid-protein interactions [41], protein-carotenoid interactions [42], protein-protein interactions [43], toxin binding [44] to give just a few examples.
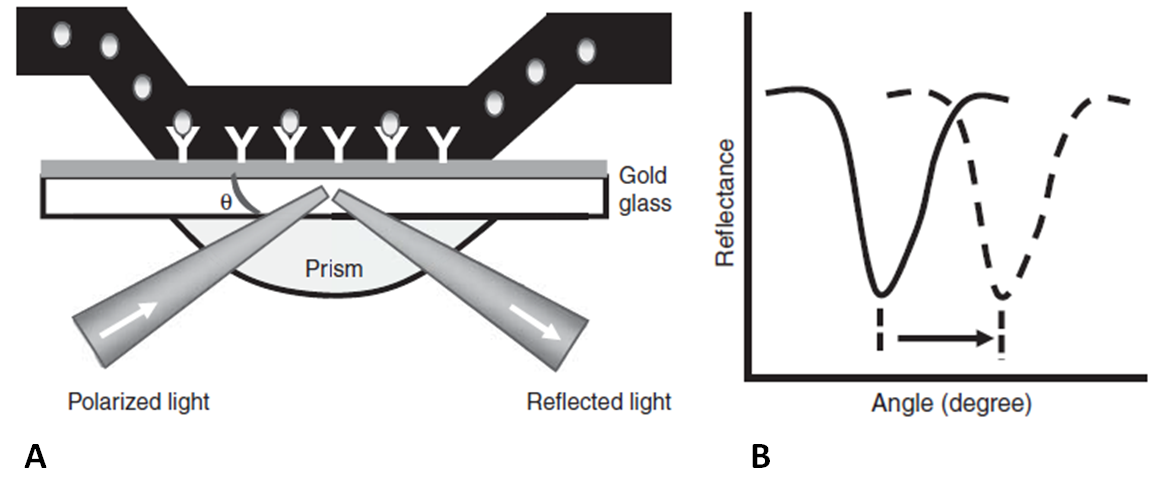
Nanofluidic Fluorescence Microscopy (NFM) applies a combination of nanofluidic-based biosensors and fluorescence microscopy. Nanofluidic immunosensor is effectively used for real-time kinetic assessment of protein/ligand interactions. In contrast to other immunoassays, NFM allows the measurement of binding kinetics in one simplified step and reduces the length of analysis [21]. In particular, this method may be used to analyze antigen-antibody binding by the direct assessment with a fluorescence microscope [45].
Similarly to SPR, plasmon-waveguide resonance method also applies surface-excited plasmon. PWR uses a polarized continuous wave laser exciting electromagnetic waves in a resonator made of a thin silver film with a layer of SiO2 and a glass prism (Figure 3). In this assay, receptors are immobilized on the outer SiO2 surface. Ligand binding changes amplitude, position and width of reflected lights. In contrast to SPR, PWR examines anisotropic optical contents of receptor-ligand complexes and differentiates mass density changes from conformational changes [46]. Thus, PWR can be applied to analyze the changes in receptor conformation and local mass density [47]. This assay shows that distinct ligands including full agonists, partial agonists and antagonists cause distinct conformational changes in receptors. PWR has been reported to be a useful tool to investigate receptor-mediated processes in real biological membranes, since it has been used to study ligand binding to integral membrane proteins obtained from bacterial and mammalian cell membranes [48].
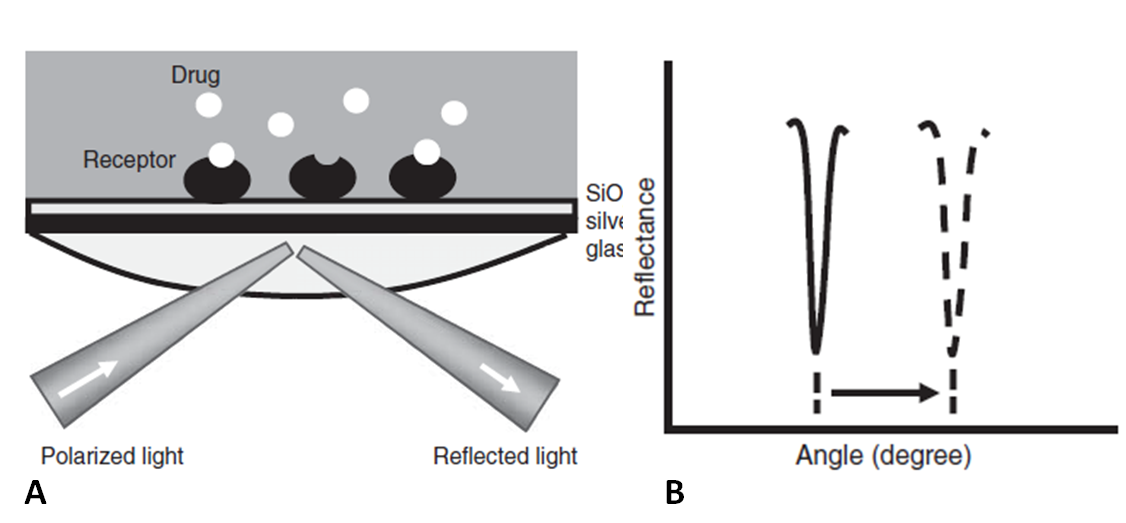
SPR imaging for affinity-based biosensors measures the binding kinetics and is related to intensity modulation and analyzes the reflectivity of monochromatic incident p-polarized light detected at a fixed angle [49]. This method studies binding events across the entire sensor surface. Besides, SPR imaging studies are often combined with microarrays to measure specific microspots [50]. SPR imaging analyzes different chemical compounds for a target of interest. In addition, this method has been used for biomarker identification, for detecting microRNA and for analysis of interactions of proteins, DNA and RNA and whole cells.
Whispering gallery microresonator (WGM) is based on wave sensing and applies different microresonators or resonant cavities having high-quality factors to limit light, which generates very sharp resonances for the light [51]. Binding of molecules to the surface of the cavity induces changes of the resonant wavelength changes. The resonant changes of light permit multiple analyses of molecules. In contrast to other label-free ligand binding assays, backscattering interferometry (BSI) has an advantage as homogenous solution-based biosensing. BSI has been used to detect the equilibrium dissociation constants for different types of receptor-ligand binding, including antigen-antibody interactions, and host-pathogen interactions [52].
Several improvements to the technique already enable enhanced device performance [53], allowing their use in complex environments [54]. Xu et al [55] recently demonstrated that microresonators might be soon biodegradable and based on biocompatible proteins. More information on the newest device in the area of biosensing and perspectives of further developments can be found in a recent review (2016) [56].
Resonant waveguide grating (RWG) applies the electromagnetic wave bound to the surface to analyze biomolecular interactions [57]. In this method, light is coupled into the thin film by diffraction leading to the generation of an evanescent wave (Figure 4). RWG uses the surface-linked electromagnetic wave to analyze interactions between ligand and receptor. In the RWG method the maximum in coupling efficiency is obtained at the resonance angle or wavelength which is a function of the local refractive index at the sensor surface. In addition, RWG is usually performed in microplate formats and is designed for affinity screening [58].
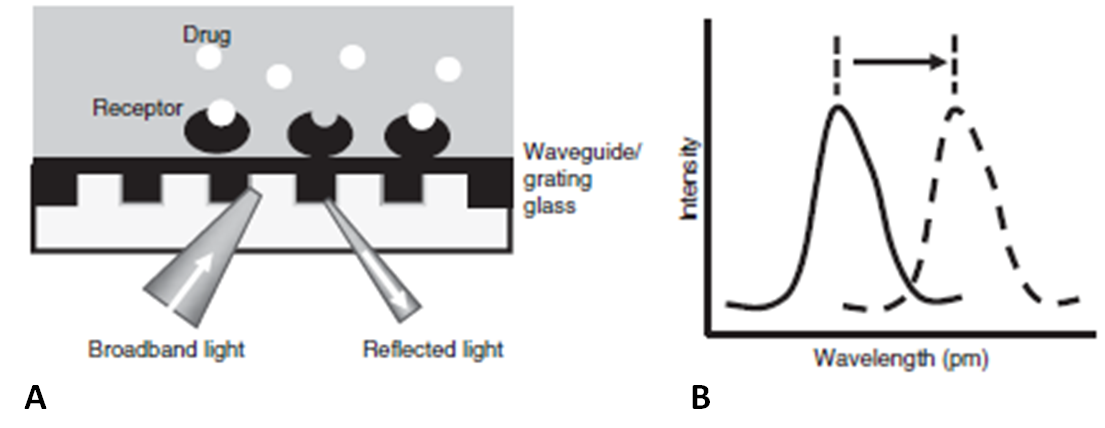
Biolayer interferometry biosensor applies a spectrometer to detect interference patterns formed by light reflected from an optical layer and a biolayer containing proteins of interest (Figure 5). These two types of light interact with each other and create a specific interference pattern. When ligands bind to the receptors, the inference pattern is altered. These changes of the interference pattern represented by specific peaks and troughs can be detected by a spectrometer. This method helps to study binding kinetics [59]. Moreover, a subtype of BIB, biolayer interferometry (BLI), can be effectively used to measure ligand binding [60] or protein-drug interaction [61]. Pastushok L et al identified a positive antibody fragment phage display clone against RAD51 protein, studied the binding of the resultant antibody fragment with RAD51, and the inhibitory effect of the antibody fragment against the binding between RAD51 and single-stranded DNA with an Octet RED384 instrument from ForteBio [62]. Silva MC et al used ForteBio Octet Red384 instrument to measure the biophysical interaction between small molecules and biotinylated tau proteins with streptavidin sensors [63]. Rice HC et al measured the binding between the GABABR1a Sushi-1 or -2 domain and sAPP peptides with a ForteBio BLItz instrument [64]. E Verschueren et al validated the interaction between AXL and IL1RL1 via BIB using an Octet Red system [40].
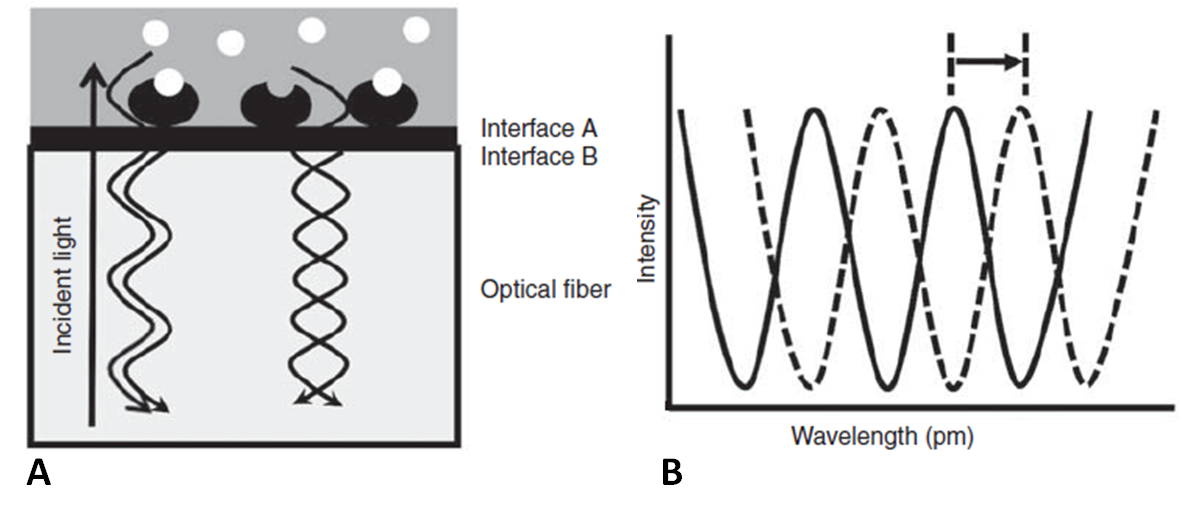
Mass spectrometry (MS) is emerging as a useful tool for the receptor-ligand binding interactions. The sensitivity of MS equipment is increasing progressively, it is possible now to detect low ligand quantities that are usually found in ligand binding assays. Some examples of its applications, are the MS binding assays developed for human dopamine [65, 66], norepinephrine, and serotonin transporters [65]. MS-based assays are often the method of choice for high-throughput drug screening and detection strategies designed to reduce the number of false positive interactions [67]. Rice HC et al purfied sAPP-binding proteins with sAPP-Fc baits from rat brain homogenates and identified these proteins through mass spectrometry [64].
Nuclear magnetic resonance (NMR) methods are well-known techniques applied to study protein–ligand complexes [68]. They include protein-observed and ligand-observed NMR methods. Protein-observed NMR methods detect the chemical shift changes of the protein resonances and therefore can be used to identify the ligand binding site. There are several ligand-based NMR methods depending on intermolecular magnetization transfer, such as saturation transfer difference spectroscopy, transferred-nuclear Overhauser effects (NOEs) spectroscopy and water–ligand observed via gradient spectroscopy experiments [3].
Ligand-observed NMR methods, which rely on intermolecular magnetization transfer, include: transferred-NOE spectroscopy (NOESY), saturation transfer difference (STD) spectroscopy and water–ligand observed via gradient spectroscopy (WaterLOGSY) [3]. In particular, transferred NOESY method analyzes spectrum on a sample of low molecular weight molecules in the presence of a substoichiometric amount of a protein. In a mixture of molecules in the presence of a protein, the molecules binding to the protein will show strong negative NOEs, whereas non-binding molecules will demonstrate very weak positive NOEs. If two ligands bind simultaneously with similar residence times on the protein surface, forming a complex. In this situation, strong negative ligand–ligand NOEs can be observed (Figure 6). In addition, when two ligand compounds bind competitively to the same binding pocket, NOE can be detected. Such NOESY cross-peaks are referred to as INPHARMA NOEs (Figure 6). INPHARMA methods were used for the detection of concentration-dependent binding of ligands to membranes containing GCPR40 [69]. Two recent reviews by Aguirre et al (2015) and Oxenoid and Chou (2016) provide great overviews of the use of both protein-observed NMR experiments and ligand-observed NMR experiments [70], as well as on the use of NMR methods in the study of ligand-induced membrane protein conformational changes [71]. Moreover, recent advances in hydrogen/deuterium exchange mass spectrometry (HDX-MS) allows for the use of such technique to identify the binding sites and the structural changes associated with protein-protein or protein-ligand interactions [72]. Rice HC et al used both protein-based and ligand-based NMR on a Bruker Avance III HD 800 MHz spectrometer equipped with a TCI cryoprobe to obtain the binding of sAPP 9mer peptide with Sushi1 domain of GABABR1a [64].
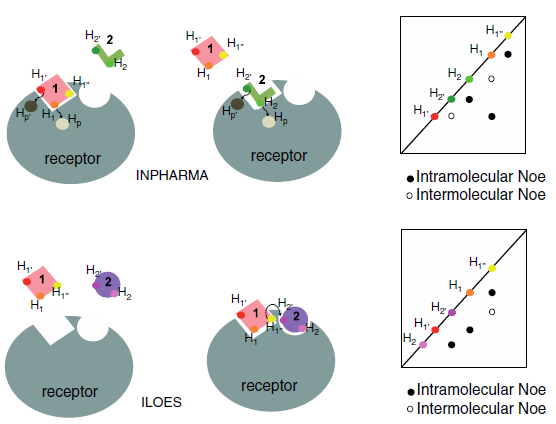
X-ray crystallography (and increasingly cryo-EM) provides a three-dimensional structure of receptor-ligand complex and is important for the target-based approach. There are several public databases such as ChEMBL, PubChem and DrugBank, which provide valuable information regarding the structures of biologically active chemicals. This information can be effectively used for structure-based ligand design. In addition, a combination of medicinal chemistry with crystallographic data on protein-ligand complexes became a powerful method. Moreover, many in silico methods such as cheminformatics [73] and virtual screening [74] are very useful for drug development.
X-ray-based methods have been used to identify the structure of GCPRs [75-77], such as histamine H1 receptor [78], sphingosine 1-phosphate S1P1 receptor [79], muscarinic M3 acetylcholine receptor [80] and other receptors [81-83]. In addition, X-ray-based assays were used to find new chemical ligands with different affinities for adenosine A2A receptor [84, 85], D3 dopamine receptors [86] and for CXCR4 receptor [87].
Thermal denaturation assays (TDA) detect the thermal denaturation of proteins by differential scanning fluorimetry (DSF) with, for example, a Quant Studio 6 real-time PCR system from Thermo Fisher [23]. In DSF, probe fluorophores are used to detect changes in the thermal denaturation patterns of proteins under different conditions, for instance in the absence or presence of ligands. Upon interaction with their specific ligands, receptors undergo structural and dynamic changes that tend to increase their thermal stability and their melting point (Tm). Such changes are detected by TDAs. So far, TDA has been used for chemical profiling of protein families and identification of novel ligands [88] or to screen proteins against a large number of putative ligand compounds in a time-effective manner and with low false-positive rates [88]. For example, Alam MM et al measured the thermostability of Plasmodium falciparum protein kinase PfCLK3 with different ligands through differential scanning fluorimetry assays using Applied Biosystems QuantStudio 6 and Protein Thermal shift software [89]. A program called Meltdown [90] can be used to ease the analysis of the thermal melt curve data obtained from DSF experiments. The program performs control checks, curve normalization, outlier rejection, and Tm estimation.
A thorough description of the DSF technique is done in the recent review of DeSantis and Reinking [91]. Also, a methodological workflow for small-scale, high-throughput thermal denaturation of recombinant proteins in the presence of SYPRO Orange dye was published recently [92].
Isothermal titration calorimetry (ITC) evaluates the binding enthalpy variation by sensing the heat caused by the binding reaction. ITC experiments allow the distinction between enthalpic and entropic contributions to binding modes. Moreover, the ITC method can be used to compare ligands with similar binding affinities by detecting differences in binding modes. In particular, ITC is very reliable in analyzing entropy-driven ligands [96]. Moreover, an enthalpic array has been recently developed and can be used for the analysis of label-free interactions. ITC has been used to study the functioning of mammalian and bacterial membrane proteins [97]. In addition, ITC was applied to investigate ligand binding to functional domains of GPCR, including chemokine receptors, C5a receptor and parathyroid receptor N-terminal domains [97]. Bhogaraju S et al measured the binding affinities of Legionella SidJ protein with either apo-calmodulin or calmodulin in ITC experiments with a MicroCal ITC200 from Malvern [98].
ITC represents the gold-standard method for understanding the thermodynamics of biomolecular binding processes. It allows for the reliable determination of the dissociation constant (KD) of only one ligand, and the detection range is limited to 1nM-100 μM. Lower KDs can only be determined by performing competition or displacement assays, if a competitive ligand is available. Krainer and Keller (2015) introduced an ITC displacement assay that allows for the simultaneous characterization of two ligands of with different affinities for the same binding site [99].
Supplier | Instruments | Num | Sample references |
---|---|---|---|
MicroCal | ITC200 | 4 | the binding of XEG1 or XLP1 with GIP1 [100], and of SGN3 extracellular domain with CIF2 [101], of RALF23 peptides with FER ectodomain [102], of sAPP fragments with GABABR1a Sushi-1 or -2 domain [64] |
VP-ITC | 1 | the binding of SGN3 extracellular domain with CIF2 at pH 5.8 [101] | |
TA Instruments | Affinity | 1 | the binding of Reck and Wnt7 peptides [103] |
Surface acoustic wave (SAW) biosensor detects and analyzes the amplitudes of mechanical acoustic waves. This method is based on a sensor, in which the surface, made from a mono-crystalline piezo-material, is excited to oscillate by acoustic waves. The phase of the acoustic waves is shifted when masses of the molecules change. The conformational changes of the proteins result in changes in the amplitude of acoustic waves, which can be detected by SAW [104]. It is established that signal induced by ligand binding is related to mass changes on the surface. SAW biosensors were shown to be effective for analysis of cell/substrate interactions using class I Major Histocompatibility Complex molecule HLA-A2 and anti-HLA monoclonal antibodies [105]. Also, SAW biosensors were applied for functional assays of olfactory receptors [106].
RWG biosensor is used for biomolecular interaction analysis. Moreover, the RWG biosensor can transform drug-receptor interactions in cells into cell phenotypic signatures named dynamic mass redistribution [107, 108]. Moreover, RWG biosensor can detect cellular response formed by many cellular events which change local mass density at the surface of the sensor. After detection, the sensor analyzes the consequences of the binding reaction. Moreover, recently developed RWG imager analyzes the consequences of ligand binding using a microfluidic perfusion scheme to control the duration of exposure to the ligand [109]. The RWG imager detects the perfusion flow in real time in order to measure the effect of ligand binding (Figure 6). In particular, RWG biosensor can be used to control the duration of agonist exposure [109]. In addition, the microfluidics can detect the duration of the functional recovery of the receptor. The RWG sensor can be used to analyze the impact of protease-activated receptor PAR1 agonists on the receptor desensitization and resensitization [110]. These studies demonstrated that the RWG sensor could be used to study the functional effects of ligand-receptor interactions in living cells.
Ligand binding assays are effectively applied for both studies aiming to understand the regulation of biological functions and the research focused on developing new drugs which are strongly based on the determination of binding affinity and efficacy. It is assumed that data of in vitro binding affinity of a ligand may provide important information about in vivo efficacy of this ligand. However, many additional aspects of ligand binding should be considered. Since ligand molecules can induce conformational changes in the target proteins, structure-based methods such as X-ray and NMR should help to identify conformational changes and new binding sites. In addition, binding kinetics should be optimized in order to maximize the therapeutic efficacy of compounds. SPR, PWR and RWG methods are effectively applied for the studies of binding kinetics [109, 110]. Thermodynamics of ligand-protein interactions can be analyzed by thermodynamic binding assays. Moreover, ligand binding should be analyzed not only using purified protein molecules but also in living cells by applying the whole cell ligand-binding methods. The detailed understanding of the complexity of ligand-receptor interactions may be achieved by applying different ligand-binding assays such as labeled, label-free, thermodynamic, structure-based and whole cell ligand-binding methods.
- Fang Y. Ligand-receptor interaction platforms and their applications for drug discovery. Expert Opin Drug Discov. 2012;7:969-88 pubmed
- Owicki J. Fluorescence polarization and anisotropy in high throughput screening: perspectives and primer. J Biomol Screen. 2000;5:297-306 pubmed
- Lieto A, Cush R, Thompson N. Ligand-receptor kinetics measured by total internal reflection with fluorescence correlation spectroscopy. Biophys J. 2003;85:3294-302 pubmed
- Handl H, Gillies R. Lanthanide-based luminescent assays for ligand-receptor interactions. Life Sci. 2005;77:361-71 pubmed
- Labaer J, Ramachandran N. Protein microarrays as tools for functional proteomics. Curr Opin Chem Biol. 2005;9:14-9 pubmed
- Dong C, Liu Z, Wang F. Radioligand saturation binding for quantitative analysis of ligand-receptor interactions. Biophys Rep. 2015;1:148-155 pubmed
- Tollin G, Salamon Z, Hruby V. Techniques: plasmon-waveguide resonance (PWR) spectroscopy as a tool to study ligand-GPCR interactions. Trends Pharmacol Sci. 2003;24:655-9 pubmed
- Alves I, Cowell S, Salamon Z, Devanathan S, Tollin G, Hruby V. Different structural states of the proteolipid membrane are produced by ligand binding to the human delta-opioid receptor as shown by plasmon-waveguide resonance spectroscopy. Mol Pharmacol. 2004;65:1248-57 pubmed
- Vollmer F, Arnold S, Braun D, Teraoka I, Libchaber A. Multiplexed DNA quantification by spectroscopic shift of two microsphere cavities. Biophys J. 2003;85:1974-9 pubmed
- Young J, Garvey J, Huang P. Glial immunoreactivity for metallothionein in the rat brain. Glia. 1991;4:602-10 pubmed
- Concepcion J, Witte K, Wartchow C, Choo S, Yao D, Persson H, et al. Label-free detection of biomolecular interactions using BioLayer interferometry for kinetic characterization. Comb Chem High Throughput Screen. 2009;12:791-800 pubmed
- Imaduwage K, Go E, Zhu Z, Desaire H. HAMS: High-Affinity Mass Spectrometry Screening. A High-Throughput Screening Method for Identifying the Tightest-Binding Lead Compounds for Target Proteins with No False Positive Identifications. J Am Soc Mass Spectrom. 2016;27:1870-1877 pubmed
- Irwin J, Shoichet B. ZINC--a free database of commercially available compounds for virtual screening. J Chem Inf Model. 2005;45:177-82 pubmed
- Lyne P. Structure-based virtual screening: an overview. Drug Discov Today. 2002;7:1047-55 pubmed
- Grimes L, Young M. Purinergic P2X receptors: structural and functional features depicted by X-ray and molecular modelling studies. Curr Med Chem. 2015;22:783-98 pubmed
- Fang Y. Label-Free Receptor Assays. Drug Discov Today Technol. 2011;7:e5-e11 pubmed
- Materials and Methods [ISSN : 2329-5139] is a unique online journal with regularly updated review articles on laboratory materials and methods. If you are interested in contributing a manuscript or suggesting a topic, please leave us feedback.
- method
- Anti-GPCR Antibodies
- Aptamers and Affimers
- Assay Development: 5 Considerations and 8 Fundamentals
- Detergents: Triton X-100, Tween-20, and More
- NMR Spectroscopy in Drug Discovery and Development
- NMR in Biomedical Research
- Nanodiscs: Membrane Protein Research in Near-Native Conditions
- Neuronal Activity Research Methods
- Protein Expression
- Protein Modification
- Protein/Peptide Tags
- Protein Purification
- Protein Quantitation
- Quantitative Bioanalysis of Proteins by Mass Spectrometry