Instrumented cardiac microphysiological devices via multimaterial three-dimensional printing
- PMID: 27775708
- PMCID: PMC5321777
- DOI: 10.1038/nmat4782
Instrumented cardiac microphysiological devices via multimaterial three-dimensional printing
Abstract
Biomedical research has relied on animal studies and conventional cell cultures for decades. Recently, microphysiological systems (MPS), also known as organs-on-chips, that recapitulate the structure and function of native tissues in vitro, have emerged as a promising alternative. However, current MPS typically lack integrated sensors and their fabrication requires multi-step lithographic processes. Here, we introduce a facile route for fabricating a new class of instrumented cardiac microphysiological devices via multimaterial three-dimensional (3D) printing. Specifically, we designed six functional inks, based on piezo-resistive, high-conductance, and biocompatible soft materials that enable integration of soft strain gauge sensors within micro-architectures that guide the self-assembly of physio-mimetic laminar cardiac tissues. We validated that these embedded sensors provide non-invasive, electronic readouts of tissue contractile stresses inside cell incubator environments. We further applied these devices to study drug responses, as well as the contractile development of human stem cell-derived laminar cardiac tissues over four weeks.
Conflict of interest statement
The authors declare no competing financial interests
Figures
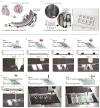
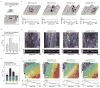
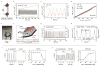
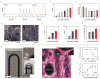
Comment in
-
Recapitulation of microtissue models connected with real-time readout systems via 3D printing technology.J Thorac Dis. 2017 Feb;9(2):233-236. doi: 10.21037/jtd.2017.02.33. J Thorac Dis. 2017. PMID: 28275467 Free PMC article. No abstract available.
Similar articles
-
Cardiac microphysiological devices with flexible thin-film sensors for higher-throughput drug screening.Lab Chip. 2017 Oct 25;17(21):3692-3703. doi: 10.1039/c7lc00740j. Lab Chip. 2017. PMID: 28976521 Free PMC article.
-
3D printed micro-scale force gauge arrays to improve human cardiac tissue maturation and enable high throughput drug testing.Acta Biomater. 2019 Sep 1;95:319-327. doi: 10.1016/j.actbio.2018.12.026. Epub 2018 Dec 19. Acta Biomater. 2019. PMID: 30576862 Free PMC article.
-
Organs-on-a-Chip.Adv Exp Med Biol. 2020;1230:27-42. doi: 10.1007/978-3-030-36588-2_3. Adv Exp Med Biol. 2020. PMID: 32285363 Review.
-
A Multimaterial Microphysiological Platform Enabled by Rapid Casting of Elastic Microwires.Adv Healthc Mater. 2019 Mar;8(5):e1801187. doi: 10.1002/adhm.201801187. Epub 2019 Feb 9. Adv Healthc Mater. 2019. PMID: 30737909
-
Printable Smart Materials and Devices: Strategies and Applications.Chem Rev. 2022 Mar 9;122(5):5144-5164. doi: 10.1021/acs.chemrev.1c00303. Epub 2021 Aug 20. Chem Rev. 2022. PMID: 34415152 Review.
Cited by
-
Designing Biomaterial Platforms for Cardiac Tissue and Disease Modeling.Adv Nanobiomed Res. 2021 Jan;1(1):2000022. doi: 10.1002/anbr.202000022. Epub 2020 Oct 16. Adv Nanobiomed Res. 2021. PMID: 33709087 Free PMC article.
-
Development of Cultured Muscles with Tendon Structures for Modular Bio-Actuators.Micromachines (Basel). 2021 Apr 1;12(4):379. doi: 10.3390/mi12040379. Micromachines (Basel). 2021. PMID: 33915803 Free PMC article.
-
Engineering Heterogeneous Tumor Models for Biomedical Applications.Adv Sci (Weinh). 2024 Jan;11(1):e2304160. doi: 10.1002/advs.202304160. Epub 2023 Nov 9. Adv Sci (Weinh). 2024. PMID: 37946674 Free PMC article. Review.
-
A Decade of Organs-on-a-Chip Emulating Human Physiology at the Microscale: A Critical Status Report on Progress in Toxicology and Pharmacology.Micromachines (Basel). 2021 Apr 21;12(5):470. doi: 10.3390/mi12050470. Micromachines (Basel). 2021. PMID: 33919242 Free PMC article. Review.
-
Motion Sensing by a Highly Sensitive Nanogold Strain Sensor in a Biomimetic 3D Environment.ACS Appl Mater Interfaces. 2024 Oct 23;16(42):56599-56610. doi: 10.1021/acsami.4c08105. Epub 2024 Sep 10. ACS Appl Mater Interfaces. 2024. PMID: 39253872 Free PMC article.
References
-
- Bhatia SN, Ingber DE. Microfluidic organs-on-chips. Nature biotechnology. 2014;32:760–772. - PubMed
-
- Mammoto A, et al. Control of lung vascular permeability and endotoxin-induced pulmonary oedema by changes in extracellular matrix mechanics. Nature communications. 2013;4:1759. - PubMed
-
- Feinberg AW, et al. Muscular thin films for building actuators and powering devices. Science. 2007;317:1366–1370. - PubMed
Publication types
MeSH terms
Grants and funding
LinkOut - more resources
Full Text Sources
Other Literature Sources