Passive Synaptic Normalization and Input Synchrony-Dependent Amplification of Cortical Feedback in Thalamocortical Neuron Dendrites
- PMID: 27030759
- PMCID: PMC4812133
- DOI: 10.1523/JNEUROSCI.3836-15.2016
Passive Synaptic Normalization and Input Synchrony-Dependent Amplification of Cortical Feedback in Thalamocortical Neuron Dendrites
Abstract
Thalamocortical neurons have thousands of synaptic connections from layer VI corticothalamic neurons distributed across their dendritic trees. Although corticothalamic synapses provide significant excitatory input, it remains unknown how different spatial and temporal input patterns are integrated by thalamocortical neurons. Using dendritic recording, 2-photon glutamate uncaging, and computational modeling, we investigated how rat dorsal lateral geniculate nucleus thalamocortical neurons integrate excitatory corticothalamic feedback. We find that unitary corticothalamic inputs produce small somatic EPSPs whose amplitudes are passively normalized and virtually independent of the site of origin within the dendritic tree. Furthermore, uncaging of MNI glutamate reveals that thalamocortical neurons have postsynaptic voltage-dependent mechanisms that can amplify integrated corticothalamic input. These mechanisms, involving NMDA receptors and T-type Ca(2+)channels, require temporally synchronous synaptic activation but not spatially coincident input patterns. In hyperpolarized thalamocortical neurons, T-type Ca(2+)channels produce nonlinear amplification of temporally synchronous inputs, whereas asynchronous inputs are not amplified. At depolarized potentials, the input-output function for synchronous synaptic input is linear but shows enhanced gain due to activity-dependent recruitment of NMDA receptors. Computer simulations reveal that EPSP amplification by T-type Ca(2+)channels and NMDA receptors occurs when synaptic inputs are either clustered onto individual dendrites or when they are distributed throughout the dendritic tree. Consequently, postsynaptic EPSP amplification mechanisms limit the "modulatory" effects of corticothalamic synaptic inputs on thalamocortical neuron membrane potential and allow these synapses to act as synchrony-dependent "drivers" of thalamocortical neuron firing. These complex thalamocortical input-output transformations significantly increase the influence of corticothalamic feedback on sensory information transfer.
Significance statement: Neurons in first-order thalamic nuclei transmit sensory information from the periphery to the cortex. However, the numerically dominant synaptic input to thalamocortical neurons comes from the cortex, which provides a strong, activity-dependent modulatory feedback influence on information flow through the thalamus. Here, we reveal how individual quantal-sized corticothalamic EPSPs propagate within thalamocortical neuron dendrites and how different spatial and temporal input patterns are integrated by these cells. We find that thalamocortical neurons have voltage- and synchrony-dependent postsynaptic mechanisms, involving NMDA receptors and T-type Ca(2+)channels that allow nonlinear amplification of integrated corticothalamic EPSPs. These mechanisms significantly increase the responsiveness of thalamocortical neurons to cortical excitatory input and broaden the "modulatory" influence exerted by corticothalamic synapses.
Keywords: NMDA receptor; T-type calcium channel; dendritic integration; passive normalization; thalamocortical.
Copyright © 2016 Connelly et al.
Figures
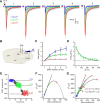
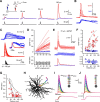
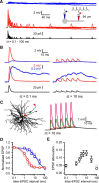
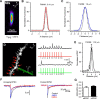
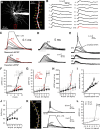
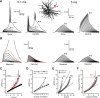
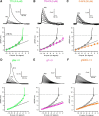
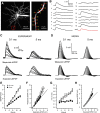
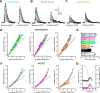
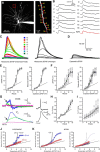
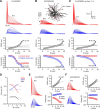
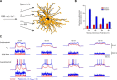
Similar articles
-
The impact of corticothalamic feedback on the output dynamics of a thalamocortical neurone model: the role of synapse location and metabotropic glutamate receptors.Neuroscience. 2003;117(1):229-39. doi: 10.1016/s0306-4522(02)00759-5. Neuroscience. 2003. PMID: 12605909
-
Two classes of excitatory synaptic responses in rat thalamic reticular neurons.J Neurophysiol. 2016 Sep 1;116(3):995-1011. doi: 10.1152/jn.01121.2015. Epub 2016 Jun 8. J Neurophysiol. 2016. PMID: 27281752 Free PMC article.
-
Anatomic, intrinsic, and synaptic properties of dorsal and ventral division neurons in rat medial geniculate body.J Neurophysiol. 1999 May;81(5):1999-2016. doi: 10.1152/jn.1999.81.5.1999. J Neurophysiol. 1999. PMID: 10322042
-
Dendritic enlightenment: using patterned two-photon uncaging to reveal the secrets of the brain's smallest dendrites.Neuron. 2006 Apr 20;50(2):180-3. doi: 10.1016/j.neuron.2006.04.011. Neuron. 2006. PMID: 16630828 Review.
-
Synaptic integration in dendritic trees.J Neurobiol. 2005 Jul;64(1):75-90. doi: 10.1002/neu.20144. J Neurobiol. 2005. PMID: 15884003 Review.
Cited by
-
Experimentally-constrained biophysical models of tonic and burst firing modes in thalamocortical neurons.PLoS Comput Biol. 2019 May 16;15(5):e1006753. doi: 10.1371/journal.pcbi.1006753. eCollection 2019 May. PLoS Comput Biol. 2019. PMID: 31095552 Free PMC article.
-
Corticothalamic Pathways From Layer 5: Emerging Roles in Computation and Pathology.Front Neural Circuits. 2021 Sep 9;15:730211. doi: 10.3389/fncir.2021.730211. eCollection 2021. Front Neural Circuits. 2021. PMID: 34566583 Free PMC article. Review.
-
Control systems theory revisited: new insights on the brain clocks of time-to-action.Front Neurosci. 2023 Jun 9;17:1171765. doi: 10.3389/fnins.2023.1171765. eCollection 2023. Front Neurosci. 2023. PMID: 37378011 Free PMC article.
-
The Cerebellar Thalamus.Cerebellum. 2019 Jun;18(3):635-648. doi: 10.1007/s12311-019-01019-3. Cerebellum. 2019. PMID: 30827014 Review.
-
NMDA Receptors Enhance the Fidelity of Synaptic Integration.eNeuro. 2021 Mar 3;8(2):ENEURO.0396-20.2020. doi: 10.1523/ENEURO.0396-20.2020. Print 2021 Mar-Apr. eNeuro. 2021. PMID: 33468538 Free PMC article.
References
Publication types
MeSH terms
Substances
Grants and funding
LinkOut - more resources
Full Text Sources
Other Literature Sources
Molecular Biology Databases
Miscellaneous