The mechanism of orientation selectivity in primary visual cortex without a functional map
- PMID: 22442071
- PMCID: PMC6621225
- DOI: 10.1523/JNEUROSCI.6284-11.2012
The mechanism of orientation selectivity in primary visual cortex without a functional map
Abstract
Neurons in primary visual cortex (V1) display substantial orientation selectivity even in species where V1 lacks an orientation map, such as in mice and rats. The mechanism underlying orientation selectivity in V1 with such a salt-and-pepper organization is unknown; it is unclear whether a connectivity that depends on feature similarity is required, or a random connectivity suffices. Here we argue for the latter. We study the response to a drifting grating of a network model of layer 2/3 with random recurrent connectivity and feedforward input from layer 4 neurons with random preferred orientations. We show that even though the total feedforward and total recurrent excitatory and inhibitory inputs all have a very weak orientation selectivity, strong selectivity emerges in the neuronal spike responses if the network operates in the balanced excitation/inhibition regime. This is because in this regime the (large) untuned components in the excitatory and inhibitory contributions approximately cancel. As a result the untuned part of the input into a neuron as well as its modulation with orientation and time all have a size comparable to the neuronal threshold. However, the tuning of the F0 and F1 components of the input are uncorrelated and the high-frequency fluctuations are not tuned. This is reflected in the subthreshold voltage response. Remarkably, due to the nonlinear voltage-firing rate transfer function, the preferred orientation of the F0 and F1 components of the spike response are highly correlated.
Figures
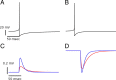
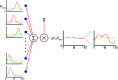
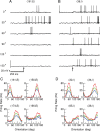
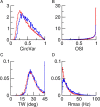
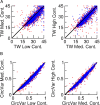
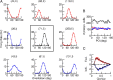
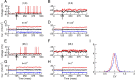
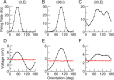
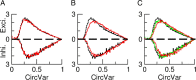
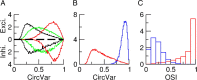
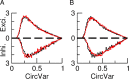
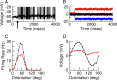
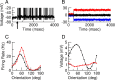
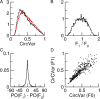
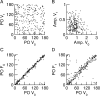
Comment in
-
Cortical selectivity through random connectivity.J Neurosci. 2012 Jul 25;32(30):10103-4. doi: 10.1523/JNEUROSCI.2463-12.2012. J Neurosci. 2012. PMID: 22836245 Free PMC article. No abstract available.
Similar articles
-
Orientation selectivity of synaptic input to neurons in mouse and cat primary visual cortex.J Neurosci. 2011 Aug 24;31(34):12339-50. doi: 10.1523/JNEUROSCI.2039-11.2011. J Neurosci. 2011. PMID: 21865476 Free PMC article.
-
Strengthening of Direction Selectivity by Broadly Tuned and Spatiotemporally Slightly Offset Inhibition in Mouse Visual Cortex.Cereb Cortex. 2015 Sep;25(9):2466-77. doi: 10.1093/cercor/bhu049. Epub 2014 Mar 20. Cereb Cortex. 2015. PMID: 24654259 Free PMC article.
-
Synaptic Basis for Differential Orientation Selectivity between Complex and Simple Cells in Mouse Visual Cortex.J Neurosci. 2015 Aug 5;35(31):11081-93. doi: 10.1523/JNEUROSCI.5246-14.2015. J Neurosci. 2015. PMID: 26245969 Free PMC article.
-
The dynamics of visual responses in the primary visual cortex.Prog Brain Res. 2007;165:21-32. doi: 10.1016/S0079-6123(06)65003-6. Prog Brain Res. 2007. PMID: 17925238 Review.
-
Dynamics of orientation selectivity in the primary visual cortex and the importance of cortical inhibition.Neuron. 2003 Jun 5;38(5):689-99. doi: 10.1016/s0896-6273(03)00332-5. Neuron. 2003. PMID: 12797955 Review.
Cited by
-
Auditory map reorganization and pitch discrimination in adult rats chronically exposed to low-level ambient noise.Front Syst Neurosci. 2012 Sep 11;6:65. doi: 10.3389/fnsys.2012.00065. eCollection 2012. Front Syst Neurosci. 2012. PMID: 22973201 Free PMC article.
-
Excitation-Inhibition Balanced Neural Networks for Fast Signal Detection.Front Comput Neurosci. 2020 Sep 3;14:79. doi: 10.3389/fncom.2020.00079. eCollection 2020. Front Comput Neurosci. 2020. PMID: 33013343 Free PMC article.
-
Short-term plasticity explains irregular persistent activity in working memory tasks.J Neurosci. 2013 Jan 2;33(1):133-49. doi: 10.1523/JNEUROSCI.3455-12.2013. J Neurosci. 2013. PMID: 23283328 Free PMC article.
-
Recurrent interactions in local cortical circuits.Nature. 2020 Mar;579(7798):256-259. doi: 10.1038/s41586-020-2062-x. Epub 2020 Mar 4. Nature. 2020. PMID: 32132709 Free PMC article.
-
Development and matching of binocular orientation preference in mouse V1.Front Syst Neurosci. 2014 Jul 24;8:128. doi: 10.3389/fnsys.2014.00128. eCollection 2014. Front Syst Neurosci. 2014. PMID: 25104927 Free PMC article.
References
-
- Anderson JS, Lampl I, Gillespie DC, Ferster D. The contribution of noise to contrast invariance of orientation tuning in cat visual cortex. Science. 2000a;290:1968–1972. - PubMed
-
- Anderson JS, Lampl I, Reichova I, Carandini M, Ferster D. Stimulus dependence of two-state fluctuations of membrane potential in cat visual cortex. Nat Neurosci. 2000b;3:617–621. - PubMed
-
- Blasdel GG, Salama G. Voltage-sensitive dyes reveal a modular organization in monkey striate cortex. Nature. 1986;321:579–585. - PubMed
-
- Bonhoeffer T, Grinvald A. Iso-orientation domains in cat visual cortex are arranged in pinwheel-like patterns. Nature. 1991;353:429–431. - PubMed
MeSH terms
LinkOut - more resources
Full Text Sources
Research Materials